|
 |
THE CEREBELLUM
One way to
fully appreciate the role of the cerebellum in normal function
is to examine those signs associated with its dysfunction. These
include muscle weakness (asthenia), a decrease in muscle tone
(hypotonia), to-and-fro movements of the eyes (nystagmus),
muscle tremor while performing a voluntary task (intention
tremor), and a general loss of muscle coordination (ataxia).
Ataxia is apparent through problems with posture and gait and is
further evidenced by dysmetria, asynergia, and adiadochokinesia.
THE
CEREBELLUM AS A COMPARATOR
The cerebellum appears to function as a comparator, at least
with respect to its role in muscle control. A sample of the
motor command from the cerebral cortex to the skeletal muscles
is relayed to the cerebellar cortex for evaluation (Fig-1). Once
the motor act begins, the cerebellar cortex begins to receive
input (via spinocerebellar tracts) from the proprioceptors in
those muscles, tendons, and joints involved in the movement. In
this way the cerebellum is in a position to compare the
actual performance of a given movement with the original
"intent" of the brain. Of course this comparing only has
functional value if the cerebellum is capable of making
adjustments when the actual performance doesn't equal the
intent. As illustrated in Fig-1, the cerebellar cortex, through
the cerebellar and brainstem nuclei, can direct corrective
action both at the cortical source through ascending pathways,
as well as at the spinal cord level through descending pathways.
It is important to recognize that this simplistic mechanism is
by no means intended to fully explain the role of the cerebellum
in motor control, but is probably a good starting point from
which to understand the cerebellar function.
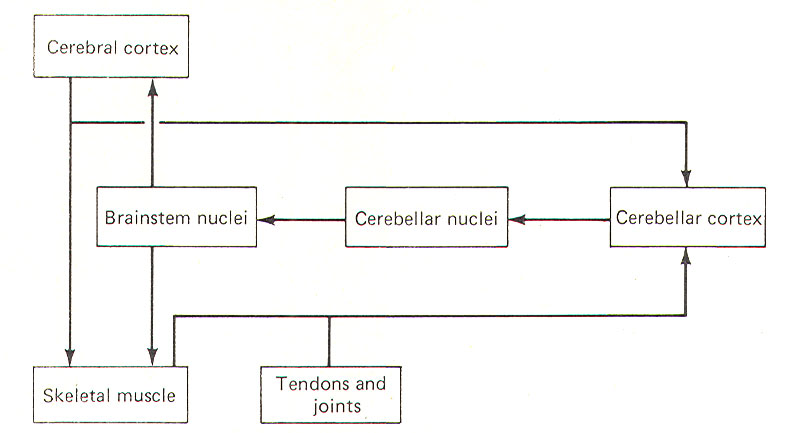 |
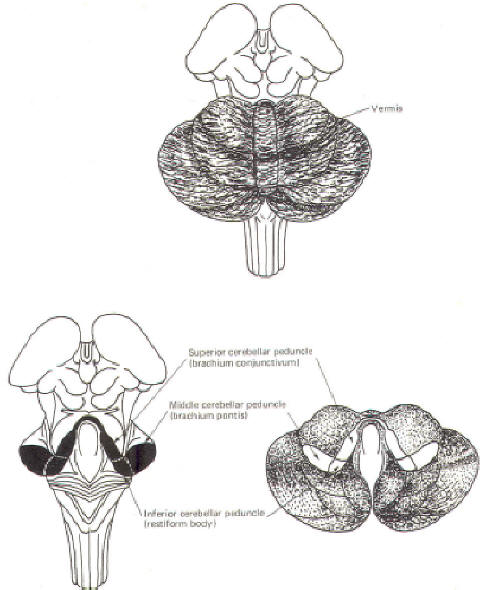 |
Fig-1 |
Fig-2 |
CEREBELLAR STRUCTURE
The cerebellum is
the largest part of the metencephalon. It lies posterior to the
pons, from which it is separated by the fourth ventricle. It is
separated from the cerebrum above by a dural covering, the
tentorium cerebelli. It weighs about 150 g in the adult male and
the ratio of cerebellar to cerebral mass is greater in the adult
than in the child.
Like the cerebrum,
the cerebellum is composed of cortical gray matter surrounding a
large area of subcortical white matter. Similarly, the
cerebellar surface is regular and grooved,
forming folia
(folds).
Some of the grooves are particularly deep, forming
fissures,
which
separate the cerebral mass into
lobules.
Also, the
cerebellum is composed of two hemispheres separated (in this
case) by the vermis
(Fig-2). The cerebellum is held firmly to the brainstem by the
cerebellar peduncles.
In
Fig-2, the peduncles are illustrated with the cerebellum
removed.
Figure-3 shows a midsagittal section of the cerebellum
through the vermis. The vermis is divided by short, deep
fissures into the
lingula, central lobule, culmen, declive, folium, tuber, pyramid,
uvula
and
nodule.
Figure-4 shows a
somewhat artificial "opened" view of the cerebellum as seen from
the rear. It can be seen here that each vermial division, with
the single exception of the lingula, is continuous laterally
with a lobule of the cerebellar hemisphere.
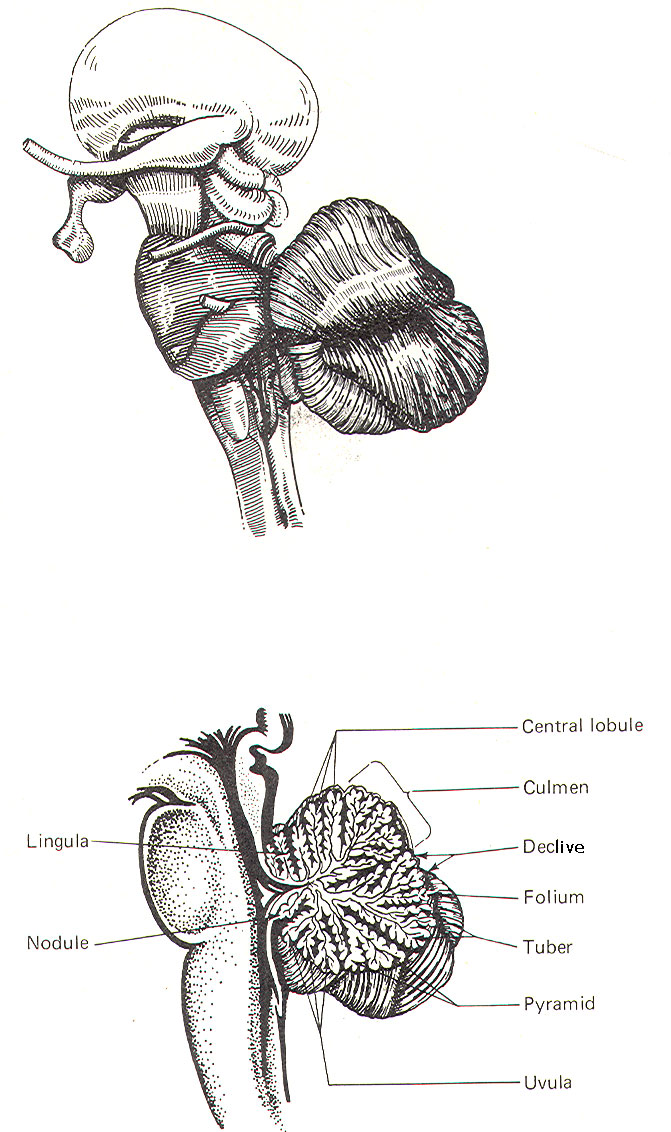 |
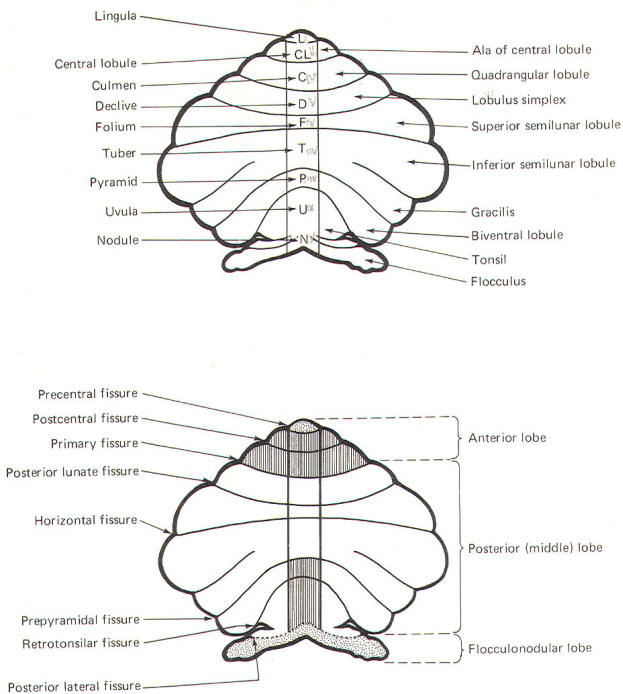 |
Fig-3 |
Fig-4 |
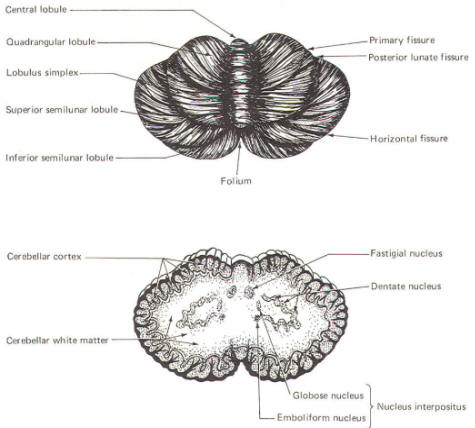 |
These include the
central lobule with the ala of the central lobule , the
culmen with the quadrangular lobule, the declive with the lobulus simplex, the folium with the superior
semilunar lobule, the tuber with the inferior semilunar
lobule and gracilis, the pyramid with the biventral
lobule, the uvula with the tonsil, and the nodule
with the flocculus.
Phylogenetically,
the lingula along with the flocculonodular lobe (nodule and
flocculi) are called the archicerebellum and represent
the cerebellum's most primitive component. Because of its close
functional relationship with the vestibular system, it is also
sometimes called the vestibulocerebellum (stippled area of Fig-4). The central lobule with its alae,
the culmen with its
quadrangles, as well as the pyramid and uvula, comprise a
somewhat more recent phylogenetic development of the cerebellum
called the
paleocerebellum
or spinocerebellum,
because of the large input it receives from the spinal cord. The
most recent addition to the cerebellum is the
neocerebellum,
composed of the
declive, folium, and tuber along with their lateral hemispheric
extensions. Also included are
the biventral lobules and tonsils. The neocerebellum is also
called the pontocerebellum
because
most of its afferent input is via the pontocerebellar
tracts.
Intracerebellar Nuclei
Another similarity between the cerebellum and the cerebrum is
the presence of nuclei in the subcortical white matter. These
are respectively called
intracerebellar nuclei
in the cerebellum
and basal nuclei
in the cerebrum. The intracerebellar nuclei are paired and
located on either side of the midline. The largest and most
lateral is the
dentate nucleus
(Fig-5). Medial
to this in order approaching the midline are the
emboliform, globose,
and fastigial nuclei.
The emboliform and
globose nuclei are known collectively as the
nucleus interpositus.
The
intercerebellar nuclei function as important relay centers
between the cerebellar cortex and other parts of the brain,
brainstem, and spinal cord. |
Fig-5 |
CEREBELLAR INPUT AND OUTPUT
Considering the importance of the cerebellum in motor control,
it is not surprising to find that there are numerous neural
pathways connecting it with the cerebral cortex, brainstem
nuclei, spinal cord proprioceptive tracts, and the vestibular
system. The information-conducting fibers entering and leaving
the cerebellum pass through the superior, middle and inferior
cerebellar peduncles.
The Inferior Cerebellar
Peduncle (Restiform Body)
The inferior
cerebellar peduncles (restiform bodies) are thick bundles of
afferent and efferent fibers which diverge as they ascend from
the posterior aspect of the medulla oblongata. As they arch
backward to enter the cerebellar hemispheres, they converge
medially and are bounded laterally by the middle cerebellar
peduncles (Fig-2). Most of the inferior cerebellar peduncular fibers are afferent, although there are some
efferent routes as well. The names of these tracts or fiber
bundles, as well as the location of the cell bodies of their
fibers and their distribution, are summarized in Table-1.
The afferent tracts
include the olivocerebellar, paraolivocerebellar,
vestibulocerebellar, reticulocerebellar, posterior
spinocerebellar, cuneocerebellar, and trigeminocerebellar. Also
included are the anterior external arcuate fibers and the striae
medullares. The efferent fibers include the cerebelloolivary,
cerebellovestibular, and cerebelloreticular tracts.
The Middle Cerebellar
Peduncle (Brachium Pontis)
The middle
cerebellar peduncles (brachie pontes), the largest of the
peduncles, are composed chiefly of fibers of the pontocerebellar
tracts. These fibers originate in the contralateral pontine
nuclei, sweep across the anterior aspect of the pons, and then
project posteriorly through the peduncles to terminate in the
cortex of the cerebellar hemispheres and vermis, except the
lingula and flocculonodular lobe.
The Superior Cerebellar
Peduncle (Brachium Conjunctivum)
The superior
cerebellar peduncles (brachia conjunctiva) emerge from the
cerebellum and ascend to form the lateral portion of the roof
of the fourth ventricle, where they enter the brainstem below
the inferior colliculi. They are bridged by the superior
medullary velum. The superior cerebellar peduncles represent the
main output route from the cerebellum, and as such, most of
their fibers are efferent. However, some afferent input
utilizes this route as well. Again, the names of these tracts or
fiber bundles and their distributions are summarized in Table-1.
The efferent
pathways include cerebellorubral, dentatothalamic, and
fastigioreticular fibers. All of them emerge from cerebellar
nuclei; the cerebellorubral fibers from the globose and
emboliform nuclei, the dentatothalamic fibers from the dentate
nucleus, and the fastigioreticular fibers from the fastigial
nucleus. They emerge together from the various nuclei to ascend
in the roof of the fourth ventricle and proceed anteriorly to
the midbrain tegmental area medial to the lateral lemniscus.
The cerebellorubral fibers cross over at this point to enter the
contralateral red nucleus. The dentatothalamic fibers also cross
over and ascend to synapse in the ventral intermediate (VI) and
ventral anterior (VA) nuclei of the thalamus. The fastigioreticular fibers enter the reticular formation of the
midbrain, pons, and medulla oblongata.
Afferent pathways include the anterior spinocerebellar and
tectocerebellar tracts. The fibers of the
anterior spinocerebellar tract originate in Clarke's column of
the spinal cord and cross in the anterior white commissure to
the lateral funiculus, where they ascend to upper pontine levels
before crossing back to enter the cerebellum through the
superior peduncle. They terminate in the hind limb region of the
cerebellar cortex. The tectocerebellar tracts emerge from the
superior and inferior colliculi on both sides, terminating in
the intermediate vermis (culmen, declive, folium, tuber,
pyramid) and the lobulus simplex. The function of the tectocerebellar tract is not known, but it is widely believed to
mediate visual and auditory reflexes.
Table-1: Cerebellar
Connections |
Tracts or fiber
bundles
|
|
Location of cell
bodies |
Inferior cerebellar peduncle |
Afferent paths
|
Olivocerebellar tract |
Lateral hemispheres and cerebellar
nucleus |
Contralateral inferior olivary nucleus
|
Paraolivocerebellar tract
|
Vermis, paravermis. and cerebellar
nucleus |
Contralateral accessory olivary nucleus
|
Vestibulocerebellar tract
|
Fastigial nucleus,
flocculonodular lobe, and uvula
|
Ipsilateral
vestibular nucleus and vestibular ganglion
|
Reticulocerebellar tract
|
Spinal region of cerebellar vermis
|
Ipsilateral lateral
reticular nucleus |
Posterior sinocerebellar tract
|
Hind limb region of cerebellar cortex
|
Ipsilateral
Clarke's column |
Trigeminocerebellar tract
|
Dentate and emboliform nucleus
|
Bilateral
principal sensory and spinal nucleus
|
Cuneocerebellar tract |
Forelimb and upper
trunk region of cerebellar cortex
|
Ipsilateral
accessory cuneate nucleus
|
Anterior exterior arcuate fibers
|
Flocculus |
Bilateral arcuate nucleus
|
Arcuatocerebellar
fibers (striae medullares)
|
Flocculus |
Bilateral arcuate nucleus
|
Efferent paths
|
Cerebelloolivary tract
|
Inferior olivary nucleus
|
|
Cerebellovestibular tract
|
Vestibular nucleus |
Fastigial nucleus
and direct axons of Purkinje cells in flocculus,
nodule, anterior and posterior vermis
|
Cerebelloreticular tract
|
Pontine and medullary reticular nucleus
|
Fastigial nucleus |
Middle cerebellar peduncle |
Afferent paths |
Pontocerebeliar
tract |
|
Contralateral
pontine nucleus
|
Superior cerebellar peduncle |
Afferent paths |
Anterior spinocerebellar tract
|
Hind limb region of cerebellar cortex
|
Ipsilateral Clarke's column |
Tectocerebellar tract |
Intermediate vermis and lobulus simplex
|
Bilateral superior and inferior colliculi |
Efferent paths |
Cerebellorubral fibers
|
Red nucleus |
Contralateral globose and emboliform
nucleus |
Dentatothalamic fibers
|
Ventral intermediate (VI) and ventral
anterior (VA) nucleus of thalamus |
Contralateral dentate nucleus |
Fastigioreticular fibers
|
Reticular nucleus of midbrain, pons, and
medulla oblongata |
Ipsilateral fastigial nucleus
|
CIRCUIT
FUNCTIONING IN THE CEREBELLAR CORTEX
In
recent years a good deal of
experimental work has helped to illuminate the roles of
individual cell types in the cerebellar cortex. Such work has
led to the development of a generally accepted model of the
interrelationships between these cells, as well as to some
elementary hypotheses of how the cerebellum performs its role in
motor control.
Unlike the cerebral
cortex, the cellular makeup of the cerebellar cortex is quite
uniform throughout. A "plug" of cortex from one area is very
much like that from any other area. Five types of excitable
cells are found in the cortex, forming three distinct layers.
Four of the five cell types are inhibitory, including Golgi
cells, stellate cells, basket cells, and Purkinje cells. The
fifth type, granular cells, represent the only excitatory cells
in the cerebellar cortex. Each of these cells, their
interactions with one another, and their relative positions in
the three cortical layers are schematically illustrated in Fig-6.
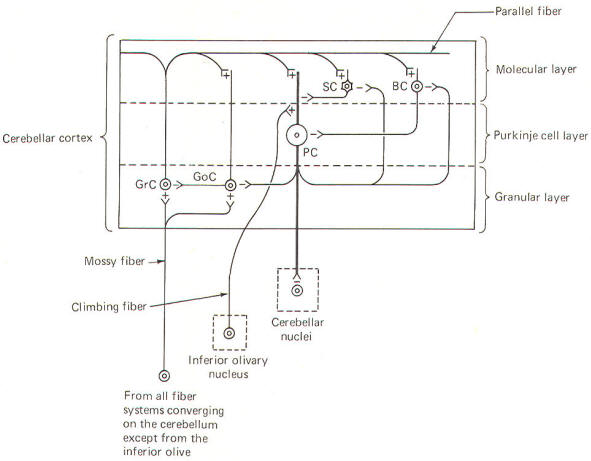 |
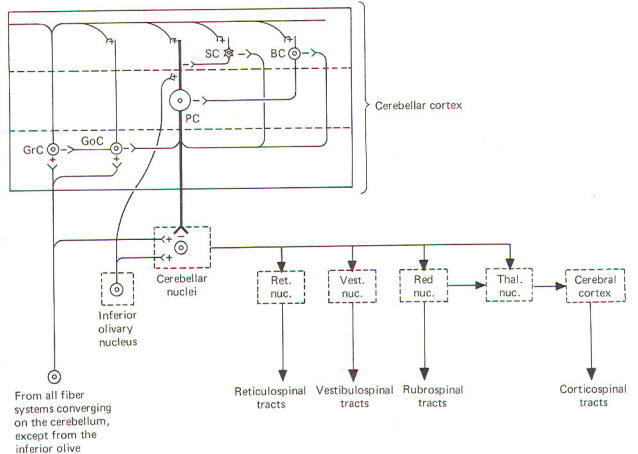 |
Fig-6 |
Fig-7 |
The deepest
(granular) layer
is made
up of granular and Golgi cells. While the cell bodies and
dendritic processes of the granular cells are located in this
layer, they project long axons up into and through the Purkinje
cell layer to ultimately reach the most superficial (molecular)
layer. Here the axons run horizontally through the molecular
layer as parallel
fibers.
Collaterals from
these parallel fibers synapse upon and excite the dendrites of
the other four cortical cell types. Golgi cells represent the
other cell type found in the granular layer. Axons from these
cells project to and inhibit the granular cells. Golgi cells
typically project a large dendritic apparatus up through the
two higher cortical layers.
The
molecular layer
contains both
stellate and basket cells. These relatively small cells are
inhibitory to the large Purkinje cells of the middle layer.
Typically, the stellate cell axonal endings are inhibitory to
the Purkinje cell dendrites, while basket cells inhibit Purkinje
cell bodies.
The
Purkinje cell layer
(middle
layer) is characterized by the presence of the Purkinje cell
bodies. These large inhibitory cells represent the only output
from the cerebellar cortex. They project flat broad dendritic
trees (Fig-9) up into the molecular layer. Most of the
Purkinje cell axons descend through the granular layer to leave
the cortex and synapse in the cerebellar nuclei. Nevertheless,
some of them from the flocculus and nodule, as well as the
anterior and posterior vermis,
project directly to the vestibular nuclei of the brainstem.
Collaterals from these axons project to and synaptically
inhibit the Golgi, stellate, and basket cells.
Two kinds of fibers
are afferent to the cerebellar cortex. These are the so-called climbing
fibers
from the inferior
olivary nucleus, and the
mossy fibers
from all other
sources afferent to the cortex. Each climbing fiber enters the
cortex and makes numerous synaptic contacts with the dendritic
tree of a single Purkinje cell. By contrast, each mossy fiber
synapses with several granular and Golgi cells. Both climbing
and mossy fibers are excitatory to the cells they synapse with.
Leaving
aside for the moment a hypothesis of how the cortical
cells integrate motor activity, let's have another look
at the cerebellum as a comparator. Recall that the
cerebellum is in a position to compare the actual
performance of a motor action with the intended command
signal and then subsequently initiate
whatever corrective action is necessary through its
efferent output. We now know that this output is a
two-link process: first from the cortex to the
cerebellar nuclei via the axons of Purkinje cells, and
then from the cerebellar nuclei through the peduncles to
the various brainstem nuclei (Fig-7).
Through
such output from the brainstem nuclei, the cerebellum
can influence motor activity both at the cortical
source as well as at the spinal cord level. Fibers leave
the cerebellum via the superior cerebellar peduncle,
projecting first to the ventral anterior (VA) and
ventral intermediate (VI) nuclei of the thalamus, to
ultimately modify cerebrocortical motor neurons through
thalamocortical projections. Similarly, through
cerebellar projections to the reticular, vestibular, and
red nuclei, the cerebellum can modify spinal cord alpha
and gamma motor neurons through the reticulospinal,
vestibulospinal, and rubrospinal tracts.
A small
basal firing rate is generally observed in the efferent
fibers from the cerebellar nuclei. This is apparently
due to the excitatory collateral input of the mossy and
climbing fibers. One can see from Fig-7 that the
cerebellar cortex is in an ideal position to modify the
firing of cerebellar nuclear fibers by varying the
firing of the inhibitory axons of Purkinje cells which
also synapse on these
neurons. Recall that the firing rate of a neuron is a
function of its central excitatory state, which is
itself a function of the "integration" of the cell's
excitatory and inhibitory input.
NEURAL "SHARPENING" OF CEREBELLAR CORTICAL INPUT
We must
recognize that even with the most recent information
concerning the functional histology of the cerebellar
cortex, little is still known about the way in which the
cortex utilizes mossy and climbing fiber input. Because
of the absence of long association fibers such as are
found in the cerebral cortex, it is assumed that small
discrete regions of the cerebellar cortex handle the
full integration of their "own" climbing and mossy
fibers, and can thereby direct appropriate output over
their own Purkinje cell axons. The cerebellar cortex has
been "mapped," and a homunculus for sensory cutaneous
stimulation is illustrated in Fig-8. The homunculus
represents points on the cerebellar cortex where
cutaneous electrical stimulation produces evoked
responses. While it is probably naive to suspect that
proprioceptors from a given muscle project to the same
region of cortex which modifies (through brainstem
nuclei and descending tracts) the motor neurons to that
same muscle, the possibility is intriguing and
undoubtedly partly true.
Evidence
suggests that the cerebellar cortex "sharpens" the input
from its afferent fibers so that it is constantly
dealing with the strongest (and presumably most
important) input at all times. A possible mechanism for
this sharpening is presented here. As Fig-9
illustrates, the dendritic trees of the Purkinje cells
are relatively flat and run in a plane transverse to the
folds of the cortical surface. The parallel fibers of
the granular cells pass through these trees much like
telephone wires on a series of poles. Because the
parallel fibers run parallel to the long axis of the
folia (folds), they cross the dendritic trees at right
angles. Consequently, when a discrete cluster of
granular cells are stimulated, a narrow strip of excited
Purkinje cells is produced down a limited length of the
folium. These same parallel fibers also make excitatory
contacts with basket. stellate, and Golgi cells. Now
recall that each of the latter are inhibitory neurons.
The stellate and basket cells are relatively small and
hence have low excitation thresholds, rendering them
easily stimulated by the parallel fibers. Their axons
are directed at more or less right angles to the
parallel fibers and make synaptic contacts with the
dendritic trees of Purkinje cells on either side of the
narrow excited strip. Because basket and stellate cells
are inhibitory, the result is the production of a narrow
inhibited zone (inhibitory surround) of Purkinje cells
on either side of the narrow excited strip. It has been
postulated that these ever-changing patterns of excited
strips flanked by the inhibitory surround provide neural
sharpening which enables the cortex to deal only with
the strongest input at all times.
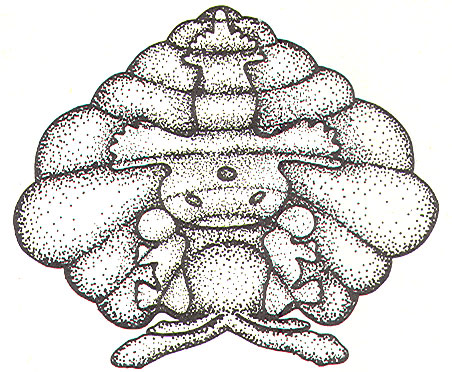 |
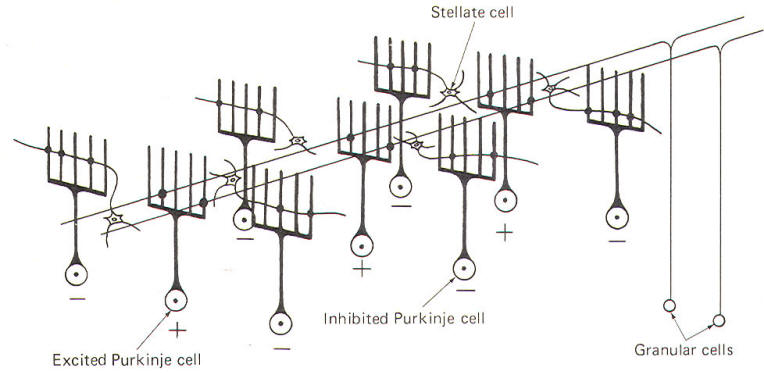 |
Fig-8 |
Fig-9 |
If
the mossy
fiber input to the cerebellar cortex is sufficient to
excite a great number of granular cells in a particular
locus, it follows that the width of the excited strip
would increase. Theoretically, this could cause the
degree of neural sharpening to decrease. Current
thinking holds that this is prevented by inhibitory
action of the Golgi cells. These cells have very
extensive dendritic projections which are not limited to
a single transverse plane like the Purkinje cells, but
rather extend through the molecular layer to share space
with the dendritic trees of as many as 10 Purkinje
cells. Now the Golgi cells aren't as easily excited as
Purkinje cells because a proportionately smaller number
of their dendritic branches receive excitatory input
from the parallel fibers. However, if the number of
granular cells firing increases, the strip of excited
Purkinje cells becomes wider also. Nevertheless, at some
point the Golgi cells will become sufficiently
stimulated by the increased number of activated
parallel fibers to inhibit the granular cells,
preventing the widening of the excitatory strip to a
point where its sharp focus is lost.
|
 |
 |
Our brain is a mystery and to understand it, you
need to be a neurosurgeon, neuroanatomist and neurophysiologist.

neurosurgery.me

Please visit this site, where daily neurosurgical activities are going
on.
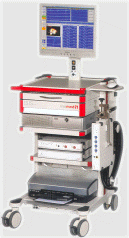
Inomed ISIS IOM System
|
|
|
|