THE SYNAPSE
Impulse
conduction in a single axon is fascinating to behold but, taken
by itself, functionally limited. The full potential of the
impulse is appreciated only by the functional changes it
produces in a postsynaptic cell. Here we will examine the events
which occur at these functional contacts known as synapses.
A PRELIMINARY OVERVIEW
Neurons
make functional contact with other neurons as well as with the
cells of skeletal muscle, cardiac muscle, smooth muscle, and
glands. The contacts neurons make with these cells are called
synapses, a term meaning "connection" coined by the English
physiologist Sherrington. The "connection" is actually an
extracellular fluid-filled synaptic cleft separating the nerve
cell membrane from the postsynaptic cell membrane (Fig-1). This
narrow cleft is typically 20 nm wide, a span sufficiently great
to bring to an abrupt halt the transmission of impulses.
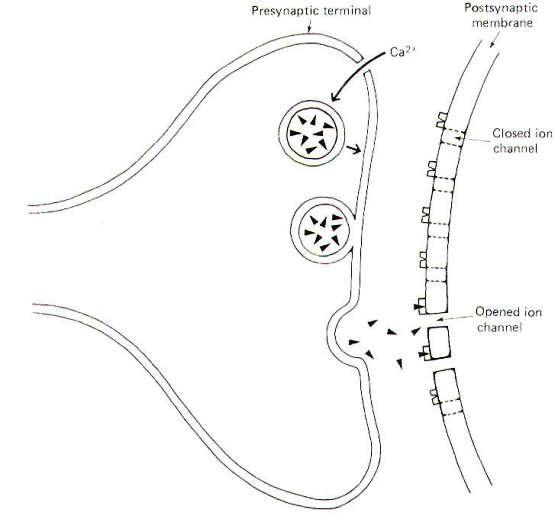 |
|
Fig-1 |
|
The signal
must bridge this cleft in order to influence the postsynaptic
cell. This is effectively produced at chemical synapses by the
release of chemical neurotransmitters from the presynaptic
terminal, which diffuse within microseconds across the cleft to
specific receptor sites on the postsynaptic cell membrane. The
neurotransmitter-receptor site interaction then causes specific
ion channels to open on the postsynaptic membrane, triggering
ionic fluxes which either depolarize or hyperpolarize the
membrane. Excitatory synapses depolarize postsynaptic membranes
while inhibitory synapses hyperpolarize them.
Depolarization of muscle cell membranes leads to contraction
while depolarization of a postsynaptic neuron leads to the
propagation of impulses on its axon. Conversely the
hyperpolarization of a muscle cell membrane prevents
contraction, while hyperpolarization of postsynaptic neurons
prevents impulse conduction.
In order to
allow presynaptic terminals to effectively control postsynaptic
cells, it is necessary to quickly inactivate the released
neurotransmitters after they have activated receptor sites,
otherwise the postsynaptic cells will continue to be stimulated
or inhibited longer than desired. Only by having the
postsynaptic response occur immediately following firing of the
presynaptic terminal, and not for prolonged periods afterwards,
can the presynaptic neuron maintain this control. Thus the
postsynaptic cell can be driven to continual action by
repetitive firing of the presynaptic neuron or brought to an
abrupt halt by the termination of presynaptic input.
Released
neurotransmitters are rendered inactive by any or all of three
means. At some synapses the transmitters are rapidly and
actively reabsorbed by the presynaptic neuron for possible
release a second time, a process called reuptake. A second means
of inactivation is by the enzymatic degradation of the
neurotransmitters by hydrolyzing enzymes which are present in
the synaptic cleft or on the postsynaptic membrane. Still a
third means of inactivation is for the transmitters to diffuse
out of the synaptic cleft and away from the receptor sites.
While the
term synapse is often used to describe all functionally active
neuron contact with receptor cells, certain additional terms are
in common use. For example, the neuron-neuron contact is called
a neuronal synapse. while the neuron-skeletal muscle cell
contact is called a neuromuscular or myoneural junction. The
contacts made by nerve cells with cardiac muscle, smooth muscle,
and gland cells are all neuroeffector junctions.
The
particular physiological response produced in a receptor cell is
determined by (1) the type of neurotransmitter released, (2) the
quantity released, (3) the type of receptor site encountered,
and (4) the particular function of the receptor cell. The
neurotransmitter acetylcholine (ACh), for example, causes an
increase in the contractility of the smooth muscle of the
stomach, while norepinephrine (NE) produces decreased activity
in this same muscle. Sufficient ACh is typically released from a
single discharge at the neuromuscular junction to produce
contraction of the skeletal muscle cell. In certain disease
conditions, however, insufficient ACh is released to reach the
excitation threshold of the cell and it does not contract.
Furthermore, receptor cells may contain different types of
receptor sites for the same neurotransmitter. For example, NE
binds with alpha receptor sites on some vascular smooth muscle
cells to produce vasoconstriction while binding with beta
receptor sites on others to produce vasodilation. Ultimately, of
course, the response capability of a receptor cell is determined
by its function in the body. Muscle cells can either contract or
relax, glandular cells can either secrete or not, and nerve
cells can be made either to conduct impulses or not.
NEUROTRANSMITTERS
Certain
synapses in invertebrates are electrically mediated rather than
dependent on chemical transmission. The clefts at such synapses
are usually narrower than those at chemical synapses. The
electrical currents associated with the impulse at the
presynaptic terminal spread across the cleft to directly
stimulate the postsynaptic membrane electrically. However, in
the overwhelming majority of mammalian and other vertebrate
synapses the cleft is too wide for electrical transmission, and
chemical transmission is required to bridge the gap.
There is
strong evidence to implicate certain chemicals as
neurotransmitters at synapses. Others, known as putative
transmitters, are also suspected to act in this way, but the
evidence supporting their participation is not as complete. It
is generally agreed that for a substance to qualify as a
neurotransmitter it must satisfy the following criteria:
1 The
substance and the enzymes necessary for its synthesis are
present in the neuron.
2 Impulses
reaching the presynaptic terminals will release the substance.
3 Systems
exist for the rapid inactivation of the substance.
4 Local
application of the substance produces changes similar to those
produced by synaptic release.
5
Drug-induced responses to both locally applied and synaptically
released substances are similar.
Acetylcholine, norepinephrine, and dopamine (DA) are chemicals
which have fulfilled all of these criteria. Nevertheless,
several additional physiological chemicals have met some but not
all of the criteria and are also suspected to function as
neurotransmitters. ACh, NE, and DA have been identified in both
the peripheral and central nervous systems while the others
listed in Table-1 are thought to operate in the CNS only.
It is
presently not known whether the enzymes necessary for the
synthesis of the various neurotransmitters are themselves
synthesized at neuronal endings since no ribosomes have been
detected in axons even with the aid of the electron microscope.
Nevertheless, the enzymes are found there. There is some
evidence to suggest that they are synthesized in the soma and
sent by axonal transport to the neuronal endings. However, it
might also be that they are synthesized at some point along the
axon by mechanisms as yet unknown. In any event
neurotransmitters are most certainly synthesized in the neuronal
endings since the rate of axonal transport is much too slow to
account for the rapid replenishment which is necessary to
prevent synaptic fatigue (neurotransmitter depletion) even in a
slowly firing neuron.
Table-1 Known and
Suspected Neurotransmitters |
|
Acetylcholine
(ACh) |
|
Norepinephrine (NE) |
|
Dopamine (DA) |
|
Prostaglandins |
|
Serotonin |
|
Histamine |
|
Glycine |
|
Aspartic acid |
|
Glutamic acid |
|
y-Aminobutyric acid (GABA) |
THE NEURONAL SYNAPSE
Much of our
knowledge about synapses is based on observations of the spinal
motor neuron. The neuronal synapse is composed of a presynaptic
terminal (PST), a synaptic cleft, and a postsynaptic membrane.
These synapses are often classified according to where they
contact the receptor neuron. Accordingly we have axodendritic,
axosomatic, and axoaxonic synapses depending on whether the PSTs
contact a dendrite, soma, or axon. Axoaxonic synapses are rare,
with dendritic and somatic contacts being the general rule.
Often hundreds to thousands of axodendritic and axosomatic
synapses will occur on a single motor neuron.
The arrival
of an impulse at the presynaptic terminal causes the release of
transmitter and its subsequent diffusion across the cleft where
it activates postsynaptic receptor sites opening specific ion
channels (Fig-1). At an excitatory synapse, ionic fluxes through
these channels tend to depolarize the membrane, while different
patterns of ionic flux hyperpolarize the membrane at inhibitory
synapses.
The Excitatory Synapse and the EPSP
Interaction
with receptor sites at excitatory synapses opens Na+
and K+ channels, thereby increasing the permeability
of the postsynaptic membrane to each of these ions. Consequently
Na+ tends to diffuse into the cell while K+
diffuses outward, each following its own chemical gradient.
However, the inward Na+ current is greater than the
outward K+ current, causing the postsynaptic membrane
to depolarize. Thus the postsynaptic membrane potential is no
longer resting and is now called an excitatory postsynaptic
potential (EPSP) (Fig-2). The potential is called excitatory
because the membrane potential is closer to the excitation
threshold than it was in the resting state.
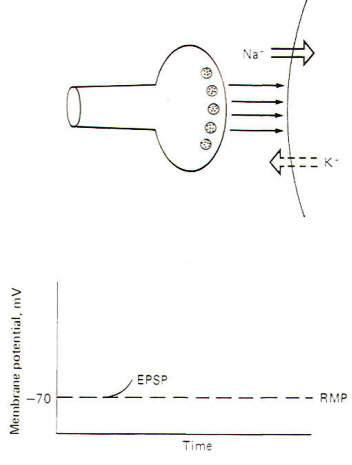 |
Fig-2 |
If the EPSP
is produced by a single, not a repetitive, volley of
transmissions across the synapse, a small EPSP called a local
response will be produced which will decay over a period of 15
ms or so to the resting state as the Na+ and K+
channels resume their normal permeabilities and neurotransmitter
is inactivated. Recall that this normal permeability has K+
diffusing outward more readily than Na+ diffuses
inward, thus repolarizing the membrane to the resting state.
The Inhibitory Synapse and the IPSP
An
inhibitory synapse produces effects just opposite to those at
the excitatory synapse. Here the action of the transmitters on
the receptor sites is to open those ionic channels which
hyperpolarize the postsynaptic membrane. Typically these are the
K+ and Cl- channels. Recall that the
chemical gradients of these two ions are such that K+
diffuses outward while Cl- diffuses in. This
combination of ionic fluxes hyperpolarizes the membrane so that
the internal potential becomes even more negative than the
resting state. Consequently an inhibitory postsynaptic potential
(IPSP) is established (Fig-3). The potential is caned inhibitory
because the membrane potential is even farther from the
excitation threshold than in the resting state.
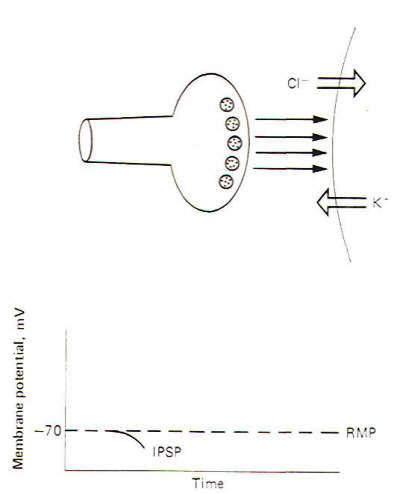 |
Fig-3 |
Electrotonic Current Spread From
Dendrites to Axon Hillock
Before an
action potential can develop in the receptor cell, the membrane
must depolarize to the excitation threshold. In the spinal cord
alpha motor neuron. this threshold is about -40 mV in the
dendrites and soma but approximately -59 mV in the initial
segment of the axon, the axon hillock. Since the resting
membrane potential in all three regions is the same
(approximately -70 mV), the axon hillock is easily the most
excitable part of the neuron as it need depolarize only 11 mV in
order to reach excitation to produce an action potential. Thus
this is the point of impulse generation in the motor neuron.
Since the
majority of synapses on the motor neuron are axodendritic and
axosomatic, one must ask the question, "How does depolarization
at a distant excitatory synapse cause depolarization of the
membrane in the axon hillock?" The answer lies in the spread of
a depolarizing electrotonic current from each synapse as it
depolarizes. An examination of the activity at a single synapse
will serve to introduce the point. When the receptors at an
excitatory synapse are activated by neurotransmitter and ion
channels open which favor a net influx of positive charges, the
postsynaptic membrane depolarizes slightly. It has been
estimated that a single synapse firing once on the motor neuron
releases enough neurotransmitter to establish an EPSP of
approximately 100 to 200 µV.
As this is obviously much too weak to reach excitation, no
action potential is generated. Further, as we pointed earlier.
this miniature EPSP will decay back to the resting membrane
potential level within 15 ms if no additional firings occur at
the synapse. Nevertheless, during the EPSP the interior of the
postsynaptic membrane is temporarily less negative than the
neuroplasm at a distance from the synapse. Accordingly, a
passive electronic (local) current spreads from the less
negative to the more negative region and out through the
adjacent membrane as a depolarizing capacitive current. The
length constant of this current is usually sufficient to reach
from even the most distant dendrite to the soma and axon
hillock. This means that while the strength of this
outwarddirected capacitive current decreases away from the
synapse. there is still some left to help depolarize the axon
hillock.
Now while
the EPSP produced by a single synapse firing once is
insufficient to produce a strong enough electrotonic current to
depolarize the axon hillock to the excitation threshold, many
separate synapses firing simultaneously, or even a single one
firing repetitively at a very high rate, are sufficient to do
so. The former pattern is spatial summation and the latter is
temporal summation. Thus the membrane potential on the axon
hillock can be depolarized to the excitation threshold and
subsequently give rise to an action potential by either spatial
or temporal summation of the synaptic EPSPs.
Spatial Summation of the Synaptic EPSPs
Spatial
summation is the establishment of a summated EPSP by the
simultaneous firing of many synapses distributed over the
dendrites and soma. If enough of them fire at the same time, the
local EPSPs will summate to produce an electrotonic current of
sufficient strength to depolarize the axon hillock to the
excitation threshold. In this way the synaptic potentials at
distant dendritic sites contribute to the production of an
action potential on the hillock through the instantaneous spread
of the electrotonic current (Fig-4). If an insufficient number
of synapses fire simultaneously, the summated EPSPs will not
reach the excitation threshold and a local response, but no
action potential will be seen. This local response is graded
while the action potential is not. This means that the amplitude
of the summated EPSPs varies directly with the number of
synapses simultaneously firing. Thus, below the excitation
threshold, increasing the number of presynaptic terminals firing
will increase the amplitude of the potential while a decrease in
the number firing will decrease it. On the other hand. if the
number of PSTs firing is sufficient to reach the excitation
threshold. a nongraded action potential will be generated.
"Nongraded" means that the amplitude of the action potential
will be the same any time enough synapses fire to reach
threshold. Even if twice this number fire the amplitude will not
change. Thus the action potential is an all-or-none response.
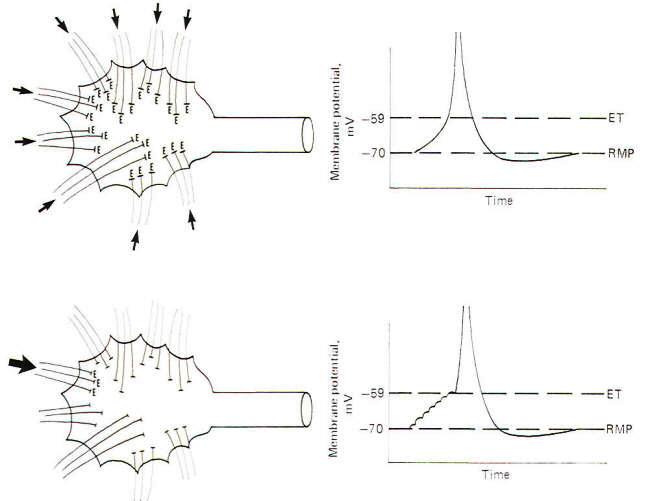 |
Fig-4 |
Once an
action potential is generated on the hillock, it self-propagates
down the length of the axon. That is, a local electrotonic
current, initiated during the reversed stage of the action
potential, travels through the axoplasm and out through the
adjacent membrane as a depolarizing capacitive current bringing
the adjacent membrane to the excitation threshold, establishing
a second action potential, and so on. A little reflection will
make it clear that the action potential, when it does occur,
will start on the axon hillock rather than on the soma or
dendrites. Even though the summated electrotonic current
generated by simultaneously firing synapses depolarizes
dendritic and somatic membranes on its way to the hillock. only
11 mV of depolarization are required for excitation here while
approximately 30 mV are necessary on the less excitable
dendrites and soma. Thus it is the first region to produce an
action potential.
One should
also be aware that the graded local response lasts longer than
the increase in Na+ conductance
gNa+
which caused it, since restoration by K+ outflow
takes a little time. This is a significant feature because it
allows the postsynaptic neuron an alternative to spatial
summation by which it can produce an action potential and a
propagated impulse. This alternative method is temporal
summation.
Temporal Summation of Synaptic EPSPs
Temporal
summation is the establishment of a summated EPSP by the
repetitive firing at a high rate of a single excitatory synapse.
Recall that the approximate 1 ms required for a single action
potential imposes an upper limit of about a thousand impulses
per second on a neuron's firing rate. Now since the EPSP from a
single synaptic firing lasts up to 15 ms. it is apparent that if
a single synapse fired repetitively at a high rate. the EPSPs
would summate, producing a greater degree of depolarization than
would be caused by a single firing. Thus the potentials are
summed over time and the process is called temporal summation.
If the depolarization produced by the temporal summation of the
synaptic EPSPs is sufficient to reach excitation. an action
potential is produced in the axon hillock. Because a single EPSP
may start to decay before the next one summates, the rise to the
excitation threshold can be pictured as a steplike progression
(Fig-4).
Synaptic Integration on the Neuron
A single
motor neuron might receive presynaptic innervation from many
hundreds or thousands of input neurons. Some of these synapses
will be excitatory, while others will be inhibitory. We have
previously described how excitatory synapses give rise to EPSPs
while IPSPs are produced at inhibitory synapses. It should be
apparent that the amplitude of the summated EPSP on the axon
hillock will be decreased by the hyperpolarizing effect of
several simultaneously firing synapses. Clearly then the state
of the membrane potential on the soma and axon hillock of the
motor neuron at any given time is determined by the number,
type, and firing frequency of its incoming synapses. Only when
this "integrated" potential exceeds the excitation threshold of
the hillock will an action potential occur.
Thus
several combinations of events exist that can produce an action
potential in the motor neuron. These are (1) a single, or at
least a very few, excitatory synapses firing simultaneously at a
high rate with no inhibitory synapses firing simultaneously. (2)
many excitatory synapses firing simultaneously at multiple
locations on the neuron with no simultaneously firing inhibitory
synapses. and (3) increasing the amplitude of the temporally or
spatially summated EPSP in order to overcome the hyperpolarizing
effect of inhibitory synapses firing simultaneously. In this
latter case, if the inhibitory synapses considered by themselves
could have produced an IPSP 3 mV more negative than resting
(i.e., -73 mV), the summated EPSP produced by the excitatory
synapses would need to be sufficiently increased to depolarize
the hillock membrane by 14 mV rather than 11 mV in order to
reach threshold and generate an action potential. A quantity of
3 mV is required to overcome the IPSP and another 11 mV to reach
-59 mV, the excitation threshold.
It
must be recognized that the membrane potential on the axon
hillock is not a simple algebraic summation of the number of
excitatory and inhibitory synapses firing at any given time. The
relative position of the synapses on the dendritic tree of the
motor neuron and the timing of their firing can have profound
effects on this "integrated" potential. For example, if a single
inhibitory synapse is located near the point where a dendrite
joins the soma while a single excitatory synapse is located near
the periphery of this same dendrite, and both are fired
simultaneously, the IPSP will have a potent effect toward
decreasing the EPSP, resulting in a seriously decreased EPSP on
the axon hillock. If, however, the position of the two synapses
is reversed with the excitatory synapse between the inhibitory
synapse and the soma, the simultaneous firing of both does not
cause much reduction in the hillock EPSP. Similarly, slight
variations in the relative timing of the firings of the synapses
can have significant effects on their ability to influence the
hillock potential.
The Central State of the Neuron and Its
Firing Rate
If all the
synapses converging on a single motor neuron were to fire just
once with the overwhelming majority being excitatory, the neuron
would depolarize to the excitation threshold of the axon hillock
and a single impulse would be generated which would travel out
along the axon to its terminals. However. if no synapses, either
excitatory or inhibitory, were to fire, the membrane potential
of the postsynaptic neuron would be considered truly resting
(Fig-5). It is possible, however, for several synapses to fire
repetitively at a low enough rate and with sufficient timing to
maintain a summated EPSP on the axon hillock several millivolts
closer to the excitation threshold than the resting state. If
this summated EPSP is 5 mV above the RMP, the neuron is said to
have a central excitatory state (CES) of 5 mV. Recognize that a
neuron which is maintaining a central excitatory state of 5 mV
is in a more excitable condition than if it were in the resting
state since it need depolarize only 6 mV more to reach threshold
(Fig-5).
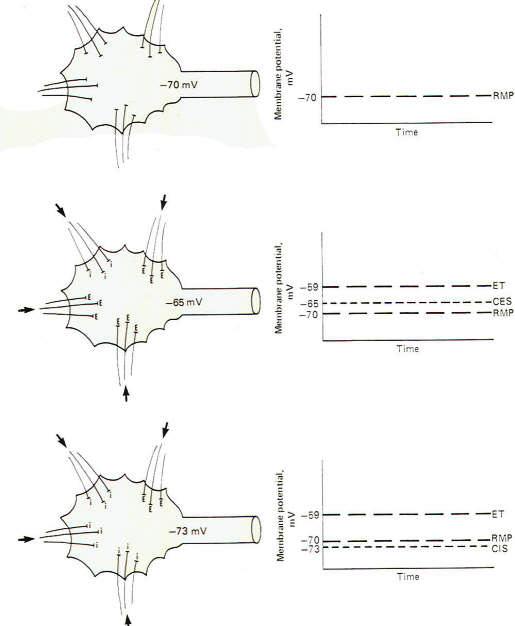 |
Fig-5 |
It is also
possible for a neuron to maintain a central inhibitory state
(CIS) by the appropriately timed repetitive firing of inhibitory
synapses. Of course in this case a greater degree of subsequent
excitatory input would be required to reach the excitation
threshold (Fig-5).
Thus. by a
steady subthreshold repetitive excitatory input, neurons can be
maintained in a "ready" condition so that they can quickly
respond to additional input and fire rapidly. One can visualize
the importance of this capability, for example, in the
activation of escape mechanisms in animal muscle systems.
Similarly, the excitability of a neuron can be decreased by the
maintenance of a CIS.
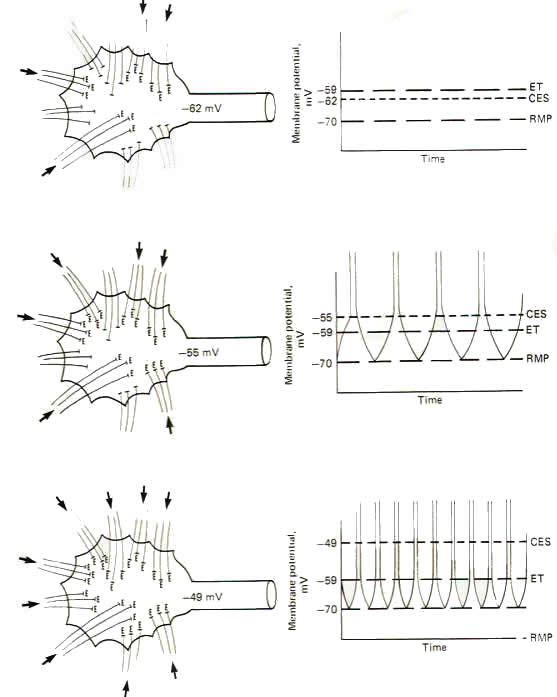 |
Fig-6 |
The upper
drawing in Fig-6 shows a motor neuron with sufficient low level
repetitive excitatory input to maintain a central excitatory
state of 8 mV. That is. the membrane potential is being held at
-62 mV. which is 8 mV above the resting state. Of course, the
motor neuron does not generate an action potential and
subsequent impulse, as the excitation threshold is not reached.
It is merely in a more excitable state. The middle and lower
drawings in Fig-6 show the effect of maintaining a central
excitatory state above the excitation threshold. In the middle
drawing. sufficient excitatory input has raised the CES to 15
mV, 4 mV above the excitation threshold. Accordingly, an action
potential will be generated on the hillock which propagates down
the axon as an impulse. Now let's look more closely at this
action potential. Following the depolarization phase in which
the reversed potential is established, the membrane needs to
return to the polarized resting state in order to be excitable
once again. This repolarization is of course caused by a strong
outward potassium current
IK+
which drives the membrane toward the potassium equilibrium
potential EK+.
However, following repolarization, the hillock membrane once
again begins to depolarize because of the steady ongoing
suprathreshold repetitive excitatory input which drives the CES
back to 15 mV. Of course as soon as the threshold point is
passed (at -59 mV), sodium conductance
gNa+
dramatically increases, producing a strong inward sodium current
INa+ and giving rise to
a second action potential. The neuron will continue to fire
impulses at a steady rate as long as the same level of CES is
maintained by the excitatory synaptic input.
Increasing
the level of this input, and hence increasing the CES, will
produce an increase in the neuron's firing rate. In the bottom
drawing in Fig-6 the CES is increased to 21 mV, maintaining the
hillock membrane potential at -49 mV. Notice that the number of
excitatory synapses firing onto the postsynaptic neuron has
increased over that shown in the middle drawing, bringing about
the increase in the CES. Notice also that the firing rate of the
second neuron has correspondingly increased because of the
increased CES. This increased firing rate is a direct result of
an increase in the speed of depolarization following each action
potential. Thus the firing rate of a continually stimulated
neuron is a function of the degree to which this stimulation
maintains a CES in excess of the neuron's excitation threshold.
Factors Affecting Synaptic Transmission
A number of
factors have been identified which influence transmission at the
synapse. These will be discussed here.
The Bell-Magendie Law and One-Way
Conduction
Whenever an
action potential is generated in the axon hillock, an impulse is
generated which travels out over the axon toward its terminal
endings. This is described as an orthodromic (running forward)
impulse. At the same time, an antidromic (running backward)
impulse is generated which spreads back over the soma, and to a
certain extent, out to the dendrites. The orthodromic impulse
carries the potential for modulating activity in the
postsynaptic neuron through synaptic transmission. That is, it
causes transmitter release and subsequent excitation or
inhibition of the postsynaptic membrane. On the other hand, the
antidromic impulse has no such potential for modulation. There
is no way for the postsynaptic membrane to communicate with the
presynaptic terminal through "backward" transmission at the
synapse. There are no vesicles releasing transmitter at the
postsynaptic membrane. Thus transmission occurs in only one
direction at the synapse, from the presynaptic terminal toward
the postsynaptic membrane. This is the Bell-Magendie law.
Synaptic Delay
The
smallest nonmyelinated type C nerve fibers conduct impulses as
slow as 0.2 m/s. whereas large myelinated type A fibers conduct
impulses at velocities up to 120 m/s. However, regardless of the
conduction velocity up to the synapse, the speed at which the
postsynaptic cell is stimulated is limited by the time required
for the events at the synapse, called synaptic delay. Because of
their ease of access, the only synapses which have been
extensively studied with regard to synaptic delay are those
incorporated in spinal reflexes. The average synaptic delay time
at these synapses is approximately 0.5 ms. This delay represents
the time it takes to release and diffuse neurotransmitter across
the synaptic cleft and for the receptor sites to become
activated. The reader should be aware that the 0.5 ms time does
not necessarily hold for all synapses, as we have no valid data
for synaptic delay times in the brain, for example. However, it
probably serves as a good first approximation.
Synaptic Fatigue
If a
presynaptic terminal fires and releases neurotransmitters faster
than it can synthesize and store new transmitter, the synapse
will soon be depleted of stored transmitter and stop
functioning. This stoppage is called synaptic fatigue. It has
been estimated that fatigue would occur within a few seconds if
resynthesis were suddenly stopped and the synapses were fired at
a high rate. There may be enough transmitter available for up to
10,000 transmissions by a single PST before it would become
totally fatigued under these conditions. Nevertheless, recognize
that under normal circumstances, synapses can fire as many as
1000 times per second for long periods of time and maintain a
reuptake and resynthesis rate sufficient to prevent fatigue.
Ca2+ and Mg2+
Concentrations and Synaptic Transmission
Up to now
we have implied that the arrival of an impulse at the
presynaptic terminal is sufficient to release neurotransmitter
from presynaptic vesicles in all circumstances. In fact, the
amount of neurotransmitter released by the arrival of the
impulse at the PST depends on the concentrations of Ca2+ and
Mg2+ in the solution bathing the terminal. If the Ca2+
concentration is reduced or the Mg2+ concentration is increased,
the amplitude of the synaptic potential is progressively
reduced.
The release of neurotransmitter is
dependent upon the entry of Ca2+ to the presynaptic terminal.
When the normal sodium and potassium currents are blocked with tetradotoxin (TTX) and tetraethylammonium (TEA), respectively,
there is a measurable inward current remaining in stimulated
axon terminals that has been shown to be totally dependent on
the concentration of external Ca2+. These data indicate that Ca2+ enters the presynaptic terminals on the arrival of an
impulse.
The
inhibitory effect of Mg2+ on transmitter release appears to be
due to its antagonistic effect on Ca2+ entry. It apparently
competes with Ca2+ for membrane sites and thus interferes with
the normal inward Ca2+ current. Thus it seems that the arrival
of the impulse at the PST causes transmitter release indirectly
by first moving Ca2+ into the terminal. Then, by some still
unknown mechanism, this causes transmitter release. Evidence
also suggests that the greatest part of the synaptic delay is
taken up by the time required for Ca2+ entry and transmitter
release.
In
experiments that exploited the fact that transmitter release can
be greatly reduced by manipulating the Ca2+ and Mg2+
concentrations, some significant features of the mechanisms of
transmitter release were discovered. For example. at very low
levels of transmitter release, the amplitude of the synaptic
potential varies on repeated observations as a multiple of some
irreducible unit size. It has been postulated that this unit
amplitude results from the release of a "quantum" of
neurotransmitter. It is likely that this quantum relates to the
number of neurotransmitter molecules in a single synaptic
vesicle. The "miniature potentials" produced by the release of
a quantum of transmitter may be the building blocks upon which
the normal synaptic potential is built when multiple vesicles
release transmitter upon the arrival of an impulse at the PST
when the concentrations of Ca2+ and Mg2+ are normal.
pH and Synaptic Transmission
Synaptic
transmission is highly pH-dependent. Increasing the pH
increases transmission while decreasing the pH decreases it.
This is particularly apparent in brain synapses where alkalosis
of 7.8 (normal. 7.4) increases excitability of neural pathways
to the point of bringing on cerebral convulsions, while a
decrease in pH to less than 7.0 decreases excitability to the
point of coma. The latter is always seen in severe uremic or
diabetic acidosis.
Drugs and Synaptic Transmission
A number of
drugs are available which can alter transmission at the neuronal
synapse. A few examples are caffeine
(found in coffee) and theophylline (found in tea). which are
known to increase synaptic excitability possibly by the
mechanism of decreasing the threshold of excitation on the
postsynaptic membrane. Strychnine is another. By its ability to
interfere with the normal spinal inhibitory input to the alpha
motor neurons, it produces hyperexcitability and muscular
convulsions. Hexamethonium and mecamylamine can both block
transmission at the synapses formed by the preganglionic and
postganglionic neurons in the ganglia of the autonomic nervous
system. In addition, a great number of agents are available
which both stimulate or depress activity in the central nervous
system. The mechanisms for their actions are largely unknown,
including whether they directly stimulate or inhibit synaptic
transmission or operate indirectly through metabolic changes in
the neurons themselves.
THE NEUROMUSCULAR
JUNCTION
In many
respects the events at the neuromuscular junction (NMJ) and the
neuronal synapse are similar. Both involve contacts between
excitable tissues. Neuron to neuron at the neuronal synapse and
neuron to skeletal muscle cell at the NMJ. Further, both
presynaptic neurons release neurotransmitter at their terminal
endings which diffuse across a narrow cleft to bind with
receptor sites on the membrane of the postsynaptic cell, opening
ion channels.
They differ
in that each vertebrate skeletal muscle cell is innervated by a
single neuron, whereas hundreds to thousands of neurons often
converge upon a single postsynaptic neuron. Another difference
lies in the fact that vertebrate neuromuscular junctions are
excitatory only. There are no inhibitory junctions. Also, ACh is
the only neurotransmitter to be identified with these junctions.
The following descriptions refer to mammalian skeletal muscle
unless otherwise indicated.
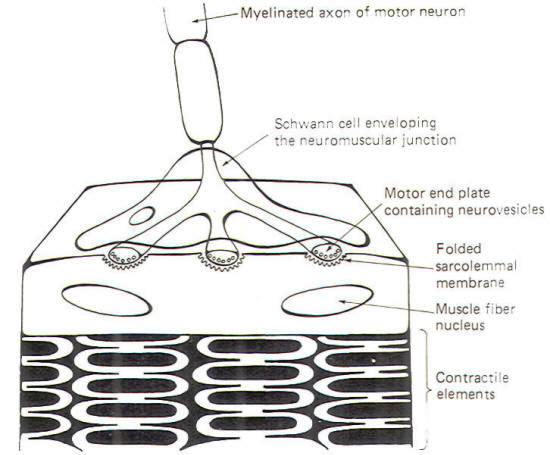 |
Fig-7 |
Skeletal
muscle cells (muscle fibers) are typically innervated by
largediameter myelinated neurons. These neurons have single
long axons which branch into filaments numbering from a very few
to several thousand. Each filament ends by forming a
neuromuscular junction with a skeletal muscle cell (Fig-7).
The neuronal filament terminates in a few flattened enlargements
known as the motor end plates. These end plates are analogous to
the presynaptic terminals in the neuronal synapse. Synaptic
vesicles containing ACh are heavily concentrated in the end
plates. The sarcolemmal membrane (muscle fiber membrane) beneath
the end plate forms a many-folded depression. The folding of the
membrane presents a greatly enlarged surface area equipped with
receptor sites responsive to the ACh released by NMJ
transmission
Activation of the Neuromuscular
Junction
Neuromuscular junction transmission begins when an impulse
reaches the motor end plate. The arrival of this impulse causes
the release of ACh into the synaptic cleft, where it diffuses
the short distance to the folded muscle fiber membrane. Here the
ACh binds with receptor sites, causing the opening of both Na+
and K+ channels. Since the ionic distribution on either side of
the sarcolemma is very similar to that which has already been
observed for nerve cell membranes. Na+ will diffuse inward while
K+ diffuses outward. Because the increase in Na+ permeability
is greater than for K+ and because Na+ is driven by both a
chemical and electrical gradient, there is a net movement of
positive charges into the cell, causing it to depolarize from
its normal resting state of approximately -85 mV. Once the
membrane starts to depolarize, it is no longer resting and its
potential is now called an end plate potential (EPP).
Action
potentials are not generated on the sarcolemmal membrane
directly beneath the end plates but rather on that portion of
the sarcolemma adjacent to the junction. Let's take a look at
how this happens. Once the EPP is established, conditions exist
for the development of an electrotonic current which spreads
away from the junction through the sarcoplasm (muscle fiber
cytoplasm) toward the still-polarized adjacent areas of the
cell. As this current spreads away from the junction, it passes
out through the adjacent sarcolemma, depolarizing it to the
excitation threshold and producing an action potential. This action
potential then propagates as an impulse over the muscle cell,
bringing about its contraction.
An
important distinction between the neuronal synapse and the
neuromuscular junction lies in the potency of a single synaptic
discharge. A single synapse discharging once is almost never
sufficient to produce an action potential in the second neuron
of a neuronal synapse. We have previously described how a great
many synapses firing simultaneously or a few firing repetitively
at a very high rate are necessary to summate EPSPs to the
excitation threshold of the postsynaptic neuron in order to
produce an action potential. By contrast, a single neuromuscular
junction firing once is almost always more than sufficient to
produce an EPP capable of generating an action potential on the
adjacent sarcolemma, bringing about impulse production and
muscle fiber contraction. In fact the arrival of a single
impulse at a single neuromuscular junction typically releases
sufficient ACh to establish an EPP four times larger than
necessary to generate the action potential. Thus we speak of a
"safety factor" of 4 at the neuromuscular junction. This may
seem like unnecessary waste of effort. However, if we consider
that each muscle fiber receives only one neuronal input, the
backup capability provided by this excess may not be out of line
after all.
As is the
case with the neuronal synapse, it is necessary to quickly
remove the neurotransmitter after each discharge in order to
keep the muscle cell from being continually stimulated, thereby
eliminating the control the nerve fiber has over the contraction
of the muscle cell it innervates. The great majority of ACh
molecules are inactivated on the spot by the action of the
enzyme acetylcholinesterase (AChE). The fraction of ACh not
inactivated in this way diffuses out of the cleft or is
reabsorbed by the end plate.
Drugs and Neuromuscular Junction
Transmission
As with the
neuronal synapse, a number of drugs are available which modify
transmission at the neuromuscular junction. Curare is the
classical competitive inhibitor at the junction. It competes
with endogenously released ACh for the receptor sites. However.
the curare-receptor site interaction does not cause
depolarization and the establishment of an EPP. It thus blocks
transmission of the signal from the nerve fiber to the muscle
cell.
While
curare is a naturally occurring drug, gallamine. benzoquinonium,
and pancuronium are synthetic curarelike compounds which block
neuromuscular transmission by similar mechanisms.
Succinylcholine and decamethonium are also neuromuscular
blocking agents but operate by a different mechanism. These
compounds produce an initial depolarization of the sarcolemmal
membrane which renders ACh incapable of producing a response in
the already depolarized membrane. Several minutes later, as the
membrane repolarizes, there is a secondary phase of decreased
receptor sensitivity to ACh.
The
neuromuscular blocking agents are primarily useful adjuncts to
anesthesia for producing muscle relaxation. They are also
useful for easing endotracheal intubation and for depressing
spontaneous contraction of respiratory muscles under certain
circumstances when artificial respirators are employed.
Neuromuscular transmission can be potentiated by the use of
drugs which inhibit the action of the enzyme AChE. Neostigmine
and physostigmine are reversible anticholinesterases. That is,
they combine with AChE, for which they have a greater affinity
than does ACh, and thus effectively tie up the enzyme so that it
cannot degrade ACh. After a few hours, neostigmine and physostigmine uncouple from the enzyme for subsequent
degradation elsewhere in the body, restoring normal function to
the neuromuscular junction. Both drugs are potent anticurare
agents as they allow ACh to build up in the synaptic cleft
giving it a favorable competitive edge over curare for the
available receptor sites. Diisopropylfluorophosphate is a
potent compound which combines irreversibly with AChE, promoting
long-term increases in neuromuscular transmission. It has had
some therapeutic application but was primarily developed as a
chemical warfare agent and is now principally of interest
because of toxicological effects associated with its use as an
insecticide. Its use produces a variety of signs and symptoms.
including muscle fasciculations, sweating, abdominal cramps,
respiratory distress, and even convulsions.
The
therapeutic application of AChE inhibitors are limited by their
lack of specificity since ACh levels are increased at ganglionic,
postganglionic, and neuromuscular receptor sites. Neostigmine
and physostigmine are primarily useful in the treatment of
myasthenia gravis and glaucoma. Both are employed for the latter
purpose, while neostigmine is commonly used for the treatment of
myasthenia. In addition to its anticholinesterase effect,
neostigmine has also been show to have a direct stimulating
effect on skeletal muscle cell receptors.
THE NEUROEFFECTOR JUNCTION
The
synapses made by autonomic nerve fibers with the cells of
cardiac muscle, smooth muscle, and glands are more varied
anatomically and chemically than are those at the neuronal
synapse previously discussed and the neuromuscular junction.
Nevertheless, these junctions are also characterized by the
presynaptic release of neurotransmitters which diffuse to
receptor sites on the effector cell membrane, producing changes
in ion permeability and initiating physiological action such as
muscle cell contraction or glandular activity.
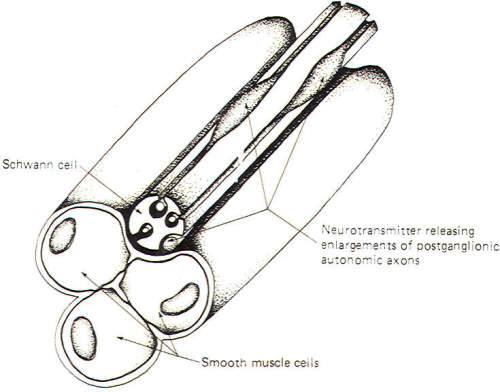 |
Fig-8 |
Fig-8
illustrates a neuroeffector junction between a nonmyelinated
postganglionic autonomic fiber and smooth muscle cells. Unlike
the skeletal muscle junction, here we have several points at
which transmitter is released to the muscle cell membrane. The
nonmyelinated axon is shown to extend out of its groove at some
places in an enveloping Schwann cell and to give rise there to
enlargements which contain neurotransmitter-releasing vesicles.
Recognize that these axons are not myelinated by the Schwann
cell but are simply enveloped in grooves formed by infoldings of
the cell. Impulse transmission along the axon causes release of
neurotransmitter at these points with the subsequent excitation
or inhibition of the muscle cells. Like the neuronal synapse
described earlier and unlike the neuromuscular junction, neuroeffector
junctions can either excite or inhibit the effector cell.
Postganglionic parasympathetic nerve fibers release ACh and give
rise to either excitatory or inhibitory effects depending on the
specific effector cell. Postganglionic sympathetic nerve fibers release either
NE or ACh. The overwhelming majority of them, however, release
NE. The effects of synaptically released NE are even more
complex. Whether it is excitatory or inhibitory depends not only
on the kind of effector cell innervated but also upon the type
of receptor site located on the cell.
Drugs and Neuroeffector Junction
Transmission
Acetylcholine, pilocarpine. and methacholine all directly
stimulate cholinergic (ACh) receptors on autonomic effector
organs. Physostigmine and neostigmine also potentiate activity
at these receptors but act indirectly by their anticholinesterase activity. On the other hand, atropine is a
potent antagonist at neuroeffector junctions by inhibiting the
action of ACh on the receptor sites. Norepinephrine, epinephrine,
isoproterenol, and phenylephrine directly stimulate adrenergic
(NE) receptors. Isoproterenol is specifically a beta receptor
stimulant (agonist), while phenylephrine is an alpha receptor
agonist. Conversely, phentolamine and phenoxybenzamine are
effective alpha antagonists that block transmission at these
junctions. while propranolol is a beta blocker. For more details
click
here!
|