SOUND AND HEARING
The ear is
the organ and sound is the sensation of hearing. A
neurophysiologist might define sound as a change in pressure
propagated through an elastic medium (typically air) which is
detected by the ear and sensed by the auditory cortex of the
brain. An understanding of the characteristics of sound waves as
well as the physics of mechanical transduction in the ear are
important correlates to the study of hearing by the nervous
apparatus. Those parameters of sound which are of particular
interest in this regard are frequency, velocity, and amplitude.
CHARACTERISTICS OF SOUND
Frequency
The
frequency f of a sound wave is equal to the number of
oscillatory cycles it makes per unit time (typically per
second). The human ear is sensitive to frequencies in the range
between 10 and 20.000 Hz (cycles per second). Actually most
people are sensitive to a narrower range between 50 and 10.000
Hz. Most speech is between 60 and 500 Hz and the ear is most
sensitive to sounds in the 1200- to 4000- Hz range. The physical
characteristics of the human hearing apparatus which favor this
very sensitive range will be explained later.
Velocity
The
velocity c of a sound wave depends on the medium through which
it travels. Typically the greater the density of the medium, the
greater the sound velocity. For example, sound travels through
air at 331 m/s, water at 1490 m/s, muscle at 1570 m/s, bone at
3360 m/s, and solids at 5000 m/s. The velocity of a sound wave
is independent of its frequency. In other words, changing its
frequency doesn't alter its velocity. If this were not the case,
low notes from a musical chord might reach the ear at a
different time than high notes from the cord, making the
appreciation of music considerably less pleasant.
The
wavelength of a sound wave is equal to its velocity divided by
its frequency and is thus expressed in distance per cycle.
l
=c/f
Amplitude
When no
sound is disturbing the air, the average pressure Po at
sea level is 1 atm. This is equivalent to 760 mmHg or 1 X 106
dyn/cm2. Sound pressure waves are superimposed on
this average pressure. Since sound waves are oscillatory, the
instantaneous absolute pressure P periodically varies above and
below the average pressure. The sound pressure amplitude
p ;
which is utilized for
calculations in sound and hearing studies, is equal to the
difference between the average and absolute pressures (Fig-1).
The sound pressure amplitude is usually expressed as dynes per
square centimeter. The threshold of human hearing for a 1000-Hz
pure tone is 2 x 10-4 dyn/cm2.
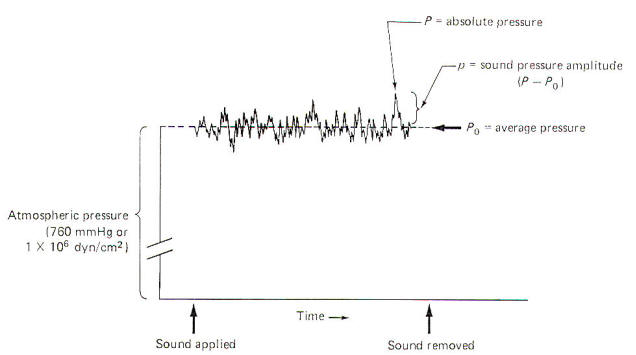 |
|
Fig-1 |
|
Sound
Pressure Level
The sound
pressure is usually expressed relative to the threshold of
hearing. This relationship, called the sound pressure level, is
measured in decibels (dB) and is calculated by the following
equation:
dB = 20 log
Pi/Po
where Pi
= actual sound pressure amplitude
Po =
reference level sound pressure amplitude (typically the hearing
threshold)
dB = sound
pressure level in decibels
A factor of
10 change in the sound pressure amplitude represents a 20-dB
change in the sound level. For example, a conversational level
of sound is about 2 x 10-1 dyn/cm2 and is
thereby 60 dB greater than the reference threshold level of 2 x
10-4 dyn/cm2. The discomfort level is about 2 x 102 dyn/cm2 (120
dB). We will deal with this equation again a little later on.
AUDITORY
STRUCTURES
The ear is
a mechanical transducer which converts the mechanical energy of
oscillating air into impulses on the cochlear portion of the
vestibulocochlear nerve (VIII). It is composed of an external,
middle, and inner portion (Fig-2).
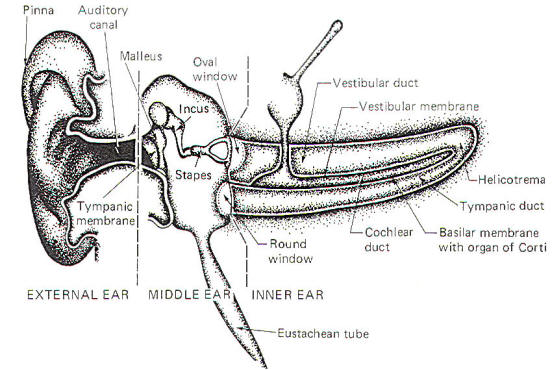 |
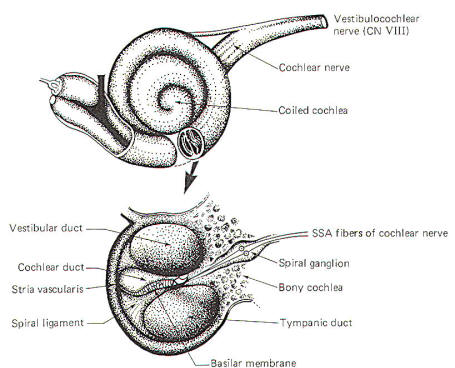 |
Fig-2 |
Fig-3 |
The
external ear is composed of the part lying outside of the head
(the pinna) and the auditory canal. The auditory canal ends at
the tympanic membrane (eardrum), which separates the external
from the middle ear. The middle ear is composed of the bony
ossicles (malleus, incus, and stapes) along with their muscles
and ligaments. It is separated from the inner ear by two thin,
flexible membranes, the oval and round windows. The pressure in
the middle ear is kept atmospheric by adjustments through the
eustachian tube which opens into the nasopharynx.
The inner
ear is composed of the cochlea, a spiral fluid-filled tube
approximately 3.5 cm long. Actually the cochlea is composed of
three fluid-filled compartments. Two of them, the vestibular
duct (scala vestibuli) and tympanic duct (scala tympani) are
filled with perilymph, a fluid with many of the same ionic
constituents as extracellular fluid. The fluid in the two ducts
is continuous only at the helicotrema at the extreme apical end
of the cochlea. The two ducts are separated from each other by a
third cochlear duct (scala media) for most of the cochlear
length (Figs-2 and 3). The cochlear duct contains a fluid
called endolymph, which is similar to intracellular fluid in
ionic concentration but is noticeably low in protein. It is
separated from the vestibular duct by the vestibular (Reissner's) membrane and from the tympanic duct by the basilar membrane.
Fixed on the basilar membrane is the mechanosensitive portion of
the cochlea, the organ of Corti (Figs-3 and 4).
Figure-3
illustrates the cochlea in its normal coiled form. The cochlea
makes 2.5 turns in forming its coil. The bottom illustration in
Fig-3 shows a cross section of the cochlea, clearly
illustrating each of the three ducts. The basilar membrane
separating the cochlear from the tympanic duct is narrow (0.04
mm) at its base near the oval window and becomes progressively
wider (0.5 mm) at its apex near the helicotrema. The basilar
membrane is attached to the outer wall of the cochlea by the
fibers of the spiral ligament. Also on the outer wall of the
cochlear duct is a secretory structure called the stria
vascularis. Centrally the membrane is attached to a bony
protuberance of the central pillar.
THE ORGAN
OF CORTI
The organ
of Corti, a sensitive structure resting on the basilar membrane,
is responsible for converting mechanical oscillations of sound
into impulses on the cochlear nerve. Because of its unique
position, the organ of Corti is particularly sensitive to
vibrations of the basilar membrane. A stiff but flexible arch of
Corti supports a reticular membrane which is penetrated by hair
cells. The internal and external spiral tunnels formed by this
arch contain a fluid very much like perilymph (Fig-4).
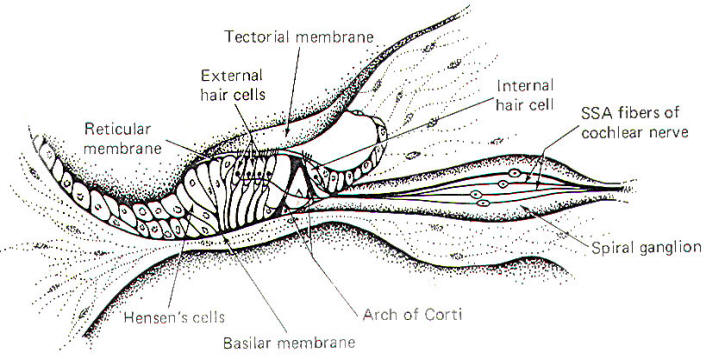 |
|
Fig-4 |
|
A tectorial
membrane makes contact with the individual hair processes of the
hair cells. The inner hair cells have 40 to 60 hairs per cell,
while the outer hair cells have as many as 80 to 100. While
the hairs of the outer cells actually touch the tectorial
membrane in the resting state, those of the inner cells
apparently contact the membrane only during part of the
oscillatory cycle of the basilar membrane. The peripheral
endings of SSA fibers of the cochlear neurons make contact with
both inner and outer hair cells. Typically one nerve fiber will
innervate a single inner hair cell, whereas a single nerve fiber
may innervate up to five or ten outer hair cells. The
significance of this innervation pattern is not understood.
As the
stapes move in and out with the vibrations of middle ear, the
oval window and perilymph of the vestibular duct are also set
into oscillation. These vibrations are, in turn, transmitted to
the endolymph of the cochlear duct through the vestibular
membrane. Movement in the cochlear duct, in turn, sets the
basilar membrane and organ of Corti into motion. The pressure
waves are ultimately absorbed by the perilymph of the tympanic
duct and damped at the round window.
Oscillations in the Basilar Membrane
Oscillations in the air are converted into oscillations in the
ossicles and ultimately into oscillations of the fluids in the
cochlea. The traveling waves which are set up on the basilar
membrane near the oval window move outward over the membrane
toward its apex near the helicotrema. Each area of the basilar
membrane has a natural frequency or resonant point where it
responds with maximum amplitude to the passage of the traveling
wave. High-frequency sounds cause maximum oscillation of the
membrane near the base and then quickly die out. Low-frequency
sounds, on the other hand, cause the membrane to oscillate
throughout its entire length but with the greatest amplitude
near the apex (Fig-5). The natural frequency or resonant
point of the basilar membrane decreases steadily from base to
apex.
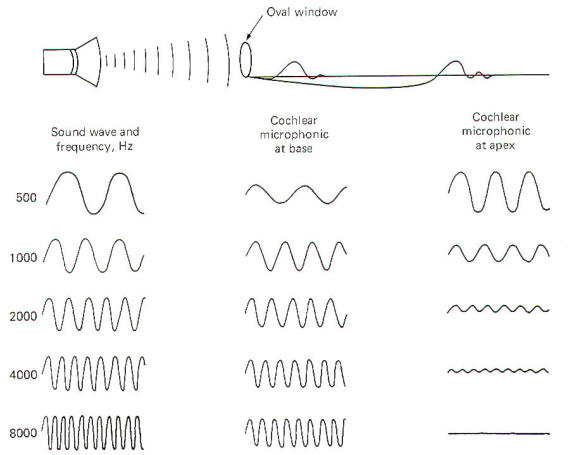 |
|
Fig-5 |
|
Hair Cell
Stimulation
The organ
of Corti hair cell is mechanosensitive. That is, it responds to
the mechanical displacement of its hairs. A stimulated hair cell
can initiate impulses in cochlear nerve fibers because the
fibers end in tufts around the base of the hair cells. However, the mechanism by which an excited hair cell stimulates and
produces impulses in the nerve fibers is still uncertain. To
examine this process, let's begin by describing changes in the
electrical activity of the cochlea in
response to sound.
The stria
vascularis secretes K+ ions into the endolymph of the cochlear duct. This
contributes to the establishment of an electrical potential
across those membranes separating endolymph from perilymph. It's
called the endocochlear potential and is typically about 80 mV
with the endolymph positive relative to the perilymph. In
addition, there is a potential difference across the membrane of
the hair cell itself with the inside about 80 mV negative with
respect to the outside. Thus there is a total potential
difference of approximately 160 mV between the endolymph in
contact with the hair cells and the cytoplasm
of the cells.
When the
basilar membrane is stimulated by sound pressure waves propagated
through the cochlear fluids, it alternately moves up and down in
response to the frequency of the sound wave. Even though this
displacement is small (about 3
µm
at the resonant point), it is sufficient to
excite the hair cells by altering the potential difference
across their membranes. As the basilar membrane goes through its
upward half-cycle, the reticular membrane with its hair cells
moves upward and backward. Alternatively, as the membrane goes
through its second half-cycle it moves downward and forward.
Because the hairs are in contact with the tectorial membrane.
they bend one way on the up cycle and the opposite way on the
downward cycle.
If a
recording electrode is placed in the endolymph of the cochlear
duct and a reference electrode placed in the perilymph, an
oscillating potential called the cochlear microphonic potential
(CMP) can be recorded when a sound is presented to the ear. This
potential has both a positive and negative component. As might
be expected, low-frequency sounds produce higher amplitude CMPs
near the apex of the basilar membrane, while those produced by
highfrequency sounds are larger near the base (Fig-5). If
the cochlear microphonic potential plays any role in impulse
production in the cochlear nerve fibers, it has not yet been
established.
The
positive and negative components of the CMP vary with the upward and
downward movements of the basilar membrane. As the membrane
moves upward, the hair cell membranes are thought to depolarize
and impulses are generated in the cochlear nerve fibers tufted
around their bases. Alternately, as the basilar membrane moves
downward the hair cell membranes hyperpolarize, decreasing
impulse production in the nerve fibers. When no sound is
presented to the ear, the basilar membrane is quiet.
Nevertheless, there is a small but basal firing rate of about 50
impulses per second on the nerve fibers which alternately
increases and decreases during oscillations of the membrane. A
single cochlear
nerve fiber has a maximum firing rate of about 1000 impulses per
second
SOUND
AMPLIFICATION THROUGH THE OUTER AND MIDDLE EAR
A sound
wave approaching the ear must displace the tympanic membrane,
the ossicles, and the fluid of the vestibular duct before it can
displace the basilar membrane and organ of Corti, generating
impulses in the cochlear nerve. During this transfer from an
air pressure wave to a fluid pressure wave, the sound loses
about 40 dB at the oval window. This is due to the fact that the
vestibular perilymph has greater inertia than the air. To
compensate for this loss of intensity, the auditory canal and
the ossicular system ordinarily amplify the incoming sound
pressure wave by about 35 dB. Because of this amplification,
little or no loss in sound intensity occurs as the wave is
transferred from the air to the fluid medium. This is an example
of impedance matching. In other words, the loss in intensity due
to the fluid inertia is compensated for by an equally strong
previous amplification.
What is the
Source of the 35-dB Gain through the Outer and Middle Ear?
As an
example, assume that a 1000-Hz pure tone with a sound pressure
amplitude of 2 x 10-1 dyn/cm2 is presented to the ear. A sound
with these characteristics will be amplified about 10 dB as it
travels through the auditory canal of the outer ear and another
25 dB as it travels through the ossicular system of the middle
ear. The 10-dB gain through the auditory canal can be explained
because it behaves exactly like sound in a closed tube. The
sound pressure is about 3 times greater at the closed end than
at the open end. This translates to a 10-dB gain as follows:
dB = 20 log
(sound pressure at eardrum/sound pressure entering canal)
= 20 log (6
x 10-1 dyn/cm2 / 2 x 10-1 dyn/cm2)
= 10-dB
gain through the auditory canal
A more
complex problem is explaining the 25-dB gain through the
ossicular system as several factors are involved. Three initial
physical characteristics of the ear are required: the surface
areas of the tympanic membrane, that portion of the membrane in
contact with the manubrium, and faceplate of the stapes in
contact with the oval window.
0.66 cm2 =
surface area of tympanic membrane
0.55 cm2 =
surface area of membrane in contact with manubrium
0.032 cm2 =
surface area of faceplate of stapes
Because of
the threefold increase in amplitude gained through the auditory
canal, the sound pressure on the tympanum is 6 x 10-1 dyn/cm2.
Since only 0.55 cm2 of the membrane is actually in contact with
the manubrium of the malleus, the force produced on the malleus
can be calculated as follows:
Force (on
malleus) = area (of malleus) x pressure (on tympanum) = (0.55
cm2) (6 x 10-1 dyn/cm2)
= 3.3 x
10-1 dyn
Experimental models indicate that the vesicles have a
theoretical mechanical advantage of 1.3. Therefore, the force on
the stapes can be calculated as follows:
Force (on
stapes) = 1.3 x force (on malleus) = 1.3 (3.3 x 10-1 dyn) =4.29
x 10-1 dyn
Given that
the area of the faceplate of the stapes is 0.032 cm2 and
knowing the force on the stapes, the pressure on the oval window
can be calculated as follows:
Sound pressure (on oval window)=force
(on stapes)/area (of stapes)
= 4.29 x
10-1 dyn/0.032 cm2
= 1.34 x
101 dyn/cm2
Using the
pressure on the tympanum as the reference level, the gain
through the ossicular system can now be calculated.
dB = 20 log
pressure (on oval window) /pressure (on tympanum)
=20 log
(1.34 x 101 dyn/cm2/6 x 10-1 dyn/cm2)
= 27-dB
gain through the ossicles
The 27-dB
gain calculated above ignores friction and damping, however.
Thus the
actual recorded experimental value is closer to 25 dB.
Consequently the total gain through the outer and middle ear is
approximately 35 dB. This is certainly important as the
air-fluid interface at the oval window reflects about 99 percent
of the sound energy back to the air. This represents a 40-dB
loss in transmission and is calculated as follows:
dB = 20 log
(pressure (in perilymph)/ pressure (on oval window))
= 20 log
(1.34 x 10-3 dyn/cm2/1.34 x 10-1 dyn/cm2)
= -40-dB
loss at oval window
It is
important to realize that impedance matching is never perfect.
It is probably 50 to 90 percent perfect for sound waves in the
300- to 3000-Hz range. This allows almost full utilization of
the energy in the incoming sound wave. However, at very high and
very low frequencies, the impedance becomes higher and thus the
impedance matching becomes poorer. Consequently a higher
threshold for hearing is observed in these ranges.
The natural
resonating frequency of the ossicular system is between 700 and
1400 Hz. However, due to the action of ligaments and muscles in
the middle ear, the system is slightly damped, causing sound
waves of 1200 Hz to be transmitted through the ossicular system
with slightly greater ease than sound waves of any other
frequency.
The natural
resonating frequency of the auditory canal is about 4000 Hz and
thus selectively favors waves of this frequency. Therefore,
combining the resonating effects of the auditory canal and the ossicular
system, the best transmission of
sound waves from air to the inner ear is for sound waves in the
1200to 4000-Hz range. Transmission is not as good above and
below this range.
Pure Tone
Threshold Curve
The
threshold of hearing is a function of sound frequency and
intensity (Fig-6). Under ideal laboratory conditions, the
threshold of hearing for a 1000Hz pure tone is 2 x 10-4
dyn/cm2.
However, as the sound frequency decreases, the threshold for
hearing increases. For example, the sound intensity would have
to be 2 x 10-2 dyn/cm2 in order to just
be able to hear a 100-Hz
pure tone. This is 40 dB greater than would be required to just
hear a 1000-Hz pure tone. Notice that the most sensitive range
is between 1200 and 4000 Hz. Points on the curve represent the
threshold of hearing for each combination of frequency and
intensity. A threshold point is established when the subject
hears the tone 50 percent of the time it is presented. A 130-dB
sound is felt as well as heard and eardrum rupture is a real
possibility at 160 dB.
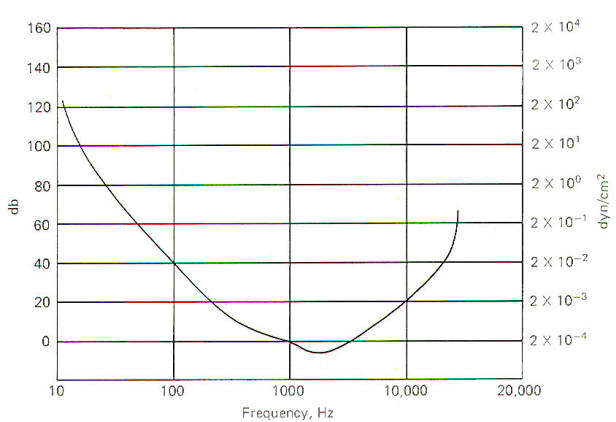 |
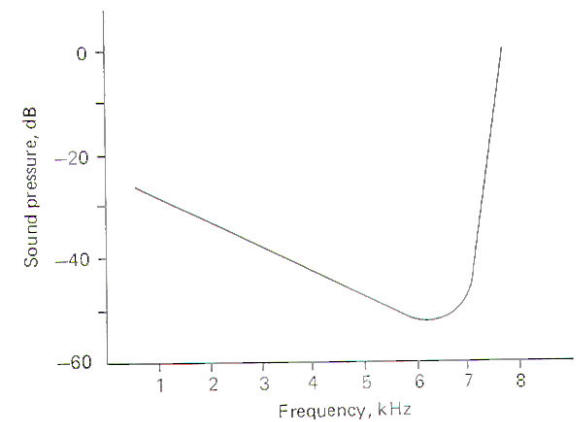 |
Fig-6 |
Fig-7 |
Response of
a Single Cochlear Nerve Fiber to Tone "Pips"
When sounds
of different frequencies are presented to the ear and responses
are recorded from a single cochlear nerve fiber, it can be seen
that the fiber has a characteristic or "best" frequency. This is
the frequency to which the fiber responds with the least
intensity (Fig-7). Notice that as the frequency is
increased or decreased from the best frequency the intensity of
the sound required to fire the fiber increases. The best
frequency of the nerve fiber illustrated is about 6.5 kHz. Thus
while it is apparently true that each area of the basilar
membrane responds maximally to a relatively narrow band of
frequencies, it will respond, although with less sensitivity,
to a broader range as well.
Auditory
Efferents and Attention Control
We
ordinarily pay very little attention to the background noise in
our immediate environment. However, if we wish to single out a
particular sound from all others we can often do it by the
conscious effort of directing our attention to that sound to the
exclusion of all others. While the mechanisms by which this is
done are not known, one intriguing possibility is based on the
discovery of auditory efferents. While their origin is largely
unknown, the possibility exists that they may function by
inhibiting the basilar membrane to tones on either side of the
desired frequency. This would in effect "sharpen" or add
"contrast" to the desired frequency range.
THE
CONSCIOUS AUDITORY PATHWAY
Special
somatic nerve fibers of the cochlear nerve conduct sound
information from the organ of Corti hair cells to the cochlear
nuclei of the brainstem. These are bipolar neurons with cell
bodies in the spiral ganglia of the cochlea with central
processes terminating in the dorsal and ventral cochlear nuclei
on the ipsilateral side of the brain stem (Fig-8). Their
fibers are tonotopically organized. This means that fibers from
each part of the basilar membrane terminate in specific areas
of the cochlear nuclei. In this way the frequency
characteristics of the membrane are preserved in the brainstem.
Most of the
second-order neurons arising in the cochlear nuclei cross over
in the trapezoid body and turn upward in the lateral lemniscus
to terminate in the inferior colliculus of the midbrain. Along
the way collaterals are sent to the nucleus of the trapezoid
body, the superior olivary nucleus, the nucleus of the lateral
lemniscus, and the brain stem reticular formation. In return,
fibers from these nuclei enter the ascending lateral lemniscus.
Fibers from the cochlear nuclei which don't cross over in the
trapezoid body ascend in the lateral lemniscus of the same
side to the ipsilateral inferior colliculus. Bilateral
connections between each inferior colliculus and each lemniscal
nucleus further convey sound information from one side to the
other. Consequently each individual lateral lemniscus conveys
sound information from both ears, which helps to explain why
damage to a lateral lemniscus produces no appreciable hearing
loss other than problems with sound localization.
From the
inferior colliculus, signals are relayed to the ipsilateral
medial geniculate body. Terminal neurons project from here to
the primary auditory area of the temporal lobe (area 41). The
adjacent cortical area (areas 22 and 42) is the auditory
association area, which is apparently necessary to make "sense"
out of the sound signals arriving at the primary area. The two
areas have extensive neural connections. The auditory pathways
are tonotopically organized all the way from the basilar
membrane, through the brain stem relay centers, to the auditory
cortex. Thus the selectivity afforded by the location of
cochlear nerve fibers and their "best frequencies" throughout
the basilar membrane are preserved in the transmission of
signals to the brain. |
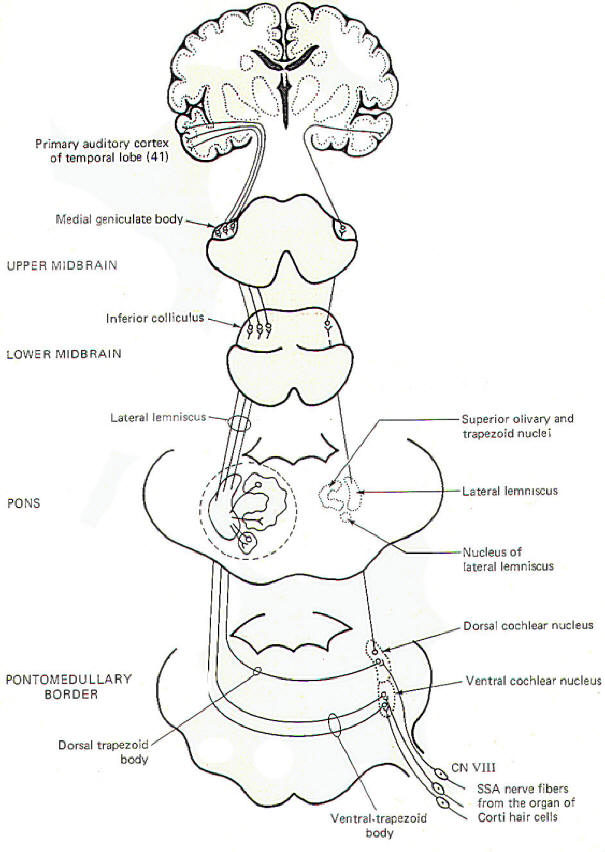 |
Fig-8 |
AUDITORY
REFLEXES
In addition
to the conscious awareness of sound, which is mediated over the
conscious pathways just described, humans are also subject to a
variety of auditory reflexes. A sudden, loud, unexpected sound
can cause reflex quickening of the pulse, increased blood
pressure, and sudden movements of the eyes, head, neck. and the
whole body. Cardiovascular and other visceral responses are
mediated over the autonomic nervous system. While the exact
relationship between the auditory system and the autonomic
nervous system is not known, the second-order neurons from the
cochlear nuclei do send some collateral fibers to the brainstem
reticular formation in addition to the other routes previously
described. The relationship between the reticular formation and
the autonomic nervous system is well documented and probably
plays some role in the cardiovascular and visceral reflexes in
response to sound.
Reflex eye
movements are mediated by input from the cochlear nuclei to both
ipsilateral and contralateral motor nuclei of cranial nerves
III, IV, and VI via the medial longitudinal fasciculus. Reflex
movements of the head, neck, and body in response to sound are
probably mediated over reflex pathways from the cochlear nuclei
to the midbrain tegmental nuclei. Descending signals over the
tectospinal tracts from these nuclei then produce the
appropriate movements.
DETERMINATION OF SOUND DIRECTION
If the head
of a person with normal hearing is fixed in a restrainer so that
it can't be moved in any direction, that person will probably
have little difficulty in determining the direction from which
a sound is coming. However, a person totally deaf in one ear
will have great difficulty in localizing the sound. The
implication is that input to both ears (binaural input) is
necessary for sound localization. Similarly, a person with
damage to the auditory cortex will also have difficulty with
sound localization. Thus it appears that central interaction of
the auditory input is also a necessary component of the process.
Time Lag
and Intensity Difference
Figure-9
illustrates how the location of a sound with respect to the head
causes a time lag and intensity difference between both ears.
When a sound source is directly in front of the head, the sound
reaches each ear at exactly the same time (no time lag) and with
the same intensity (no intensity difference). Of course the same
is true if the sound source is directly behind the head. Moving
the head slightly to the left or right creates a time lag and
intensity difference which provide clues needed for sound
localization. For example, if the sound source is directly in
front and the head is turned to the right, the sound reaches the
left ear earlier and with greater intensity than it does the
right. The intensity difference is caused primarily by the fact
that the head itself serves as somewhat of a sound shield. Of
course if the sound source were directly behind the head, moving
the head to the right would produce just the opposite effects in
time lag and intensity difference. Thus it is important to
recognize that sound localization is aided by moving the head, a
response most of us perform automatically when localizing
sounds without even thinking. Obviously, sound localization when
the head is fixed in space is much more difficult.
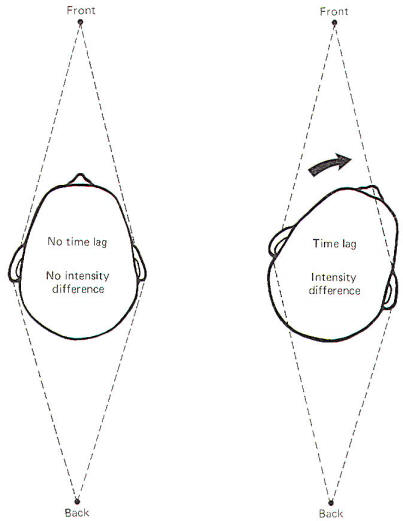 |
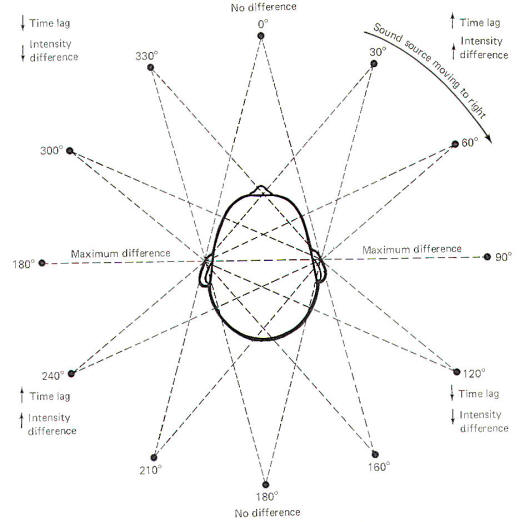 |
Fig-9 |
Fig-10 |
If a sound
source starts directly in front of the head and then moves to
the right in a circle around the head, time lag and intensity
differences will constantly be changing (Fig-10). As it
moves through the first 90° quadrant, the time lag and intensity
difference increase to a maximum. As the sound source continues
to move around the fixed head, through the remaining 270° of the
circle, predictable changes in time lag and intensity difference
occur.
Moving the
head is necessary for quadrant localization. Notice that the
time lag and intensity difference are essentially the same when
the sound source is at the 30 and 160° positions. Consequently
these two clues are not enough to tell the brain from which
direction the sound is coming. The additional clue necessary
for localization is provided by moving the head. When the head
is turned slightly to the right, there will be a decrease in the
time lag and intensity difference if the sound source is at the
30° position, and an increase in both of these parameters if the
source is in the 160° position.
The Central
Neural Mechanism for Sound Localization
The central
neural mechanisms for detecting sound direction are unknown. The
following is a hypothesis based on observed available evidence.
When recording electrodes are placed on certain projection
neurons of the medial superior olivary nucleus of the cat, a
lateral source of sound causes a net increase in activity in the
contralateral nucleus and a net decrease in the ipsilateral
nucleus. In other words, if the sound source were in the left
frontal quadrant of the cat, the right lateral lemniscal fibers
from the medial superior olivary nucleus to the inferior
colliculus would show a relative increase in firing rate, while
the left lemniscal pathway would show a decrease. This net
"weighting" of activity toward the contralateral side provides
the signal for sound lateralization.
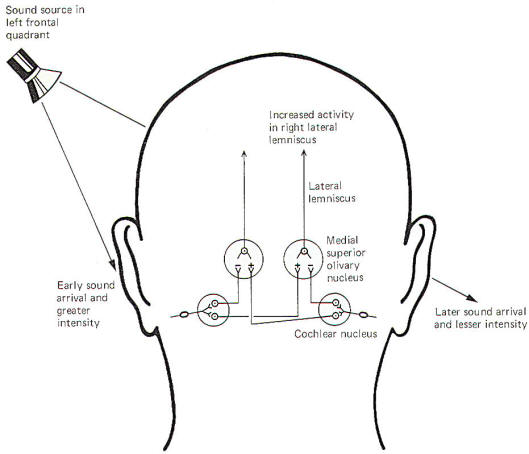 |
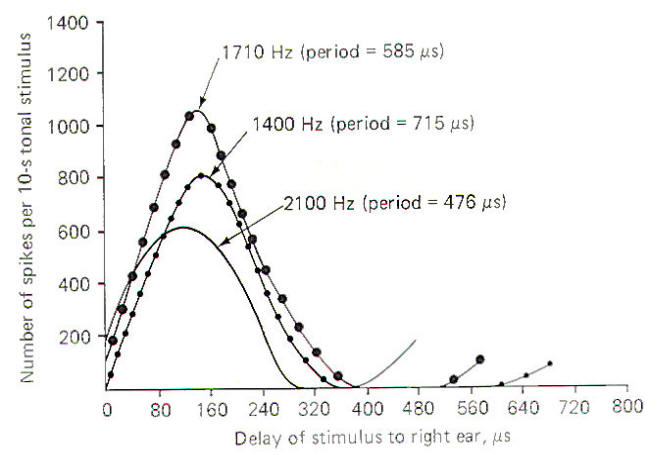 |
Fig-11 |
Fig-12 |
Figure-11 illustrates a possible mechanism for this weighting.
Certain neurons and neuronal circuits in the auditory pathway
are specifically designed for sound localization. The cochlear
nuclei project inhibitory fibers to this group of neurons in the
ipsilateral medial superior olivary nucleus and excitatory
fibers to those in the contralateral nucleus. Now if the sound
source is directly in front of the head, the sound localization
neurons in both nuclei receive identical
levels of ipsilateral inhibitory and contralateral excitatory
input, producing similar central excitatory states and firing
rates in each. Thus the brain receives the message "no
difference" over the neural pathways and interprets this as a
sound source directly in front of (or directly behind) the head.
Which of these two conditions it is can be determined by a
slight movement of the head in either direction as previously
explained. If the
sound source is moved to the left as illustrated in Fig-11,
a net weighting of activity toward the contralateral (right)
side is produced. This occurs because the sound wave reaches
the left ear a few microseconds before the right. Sound
localization neurons in the inferior colliculus respond to
different time delays, referred to as the characteristic delay
of the neuron.
Characteristic Delay and Sound Localization
Figure-12 illustrates the response of a single collicular neuron to
tones of three different frequencies in the cat when the sound
source arriving at the right ear is increasingly delayed with
respect to the left ear. Notice that as the sound source moves
from a position directly in front of the head (zero delay)
progressively to the left, the delay of stimulus to the right
ear produces a characteristic pattern of impulse firing in the
collicular neuron. The firing rate increases to a maximum at
about 140 µs and then decreases again. The delay time which
produces the greatest firing rate is the characteristic delay of
that neuron. Thus the characteristic delay in this case is 140
µs. Notice that the actual value of the maximum firing rate
varies with the frequency of the tone, but the characteristic
delay remains the same. Since different sound-delay neurons
respond maximally to different time lags, the brain interprets
the location of a sound by a combination of which sound location
neurons are maximally firing. An assumption is made that the
maximum signal a neuron can produce is an important message for
the brain with the sound-delay neurons providing the signal for
localization.
Summary of
the Neural Role in Sound Lateralization and Localization
It is
likely that the right auditory cortex recognizes that when
certain sound localization neurons fire at their maximum rate,
the sound is at a particular location in the left auditory
field. A possible mechanism for sound localization is the
following. Assume that a sound is located at a particular point
in the left auditory field so that the sound delay is 120
µs.
Those contralateral inferior collicular neurons with
characteristic delays of 120 µs will respond with their
maximum firing rate. Presumably the right auditory cortex
"recognizes" that when this particular group of neurons is
firing maximally, the sound is at a particular location in the
left auditory field. Now if the sound were to move even further
to the left, the sound delay would increase and a group of
neurons with characteristic delays equal to this new time lag
would begin to fire maximally This would signal the new position
of the sound to the auditory cortex. The left auditory cortex is
no doubt also involved in this process.
Sound
lateralization (determining whether the sound is coming from the
left or the right) is probably based on a different mechanism.
The relative weighting of activity to the contralateral lateral
lemniscus, and thus ultimately to the contralateral auditory
cortex, may be the clue for lateralization. If the brain detects
a net increase in firing in the left lateral lemniscus, it
"knows" that the sound is coming from the right auditory field,
and vice versa.
|